Geroscience and pathology: a new frontier in understanding age-related diseases
- 1Department of Public Health, Semmelweis University, Budapest, Hungary
- 2Departments of Hematology and Stem Cell Transplantation, South Pest Central Hospital, National Institute of Hematology and Infectious Diseases, Saint Ladislaus Campus, Budapest, Hungary
Geroscience, a burgeoning discipline at the intersection of aging and disease, aims to unravel the intricate relationship between the aging process and pathogenesis of age-related diseases. This paper explores the pivotal role played by geroscience in reshaping our understanding of pathology, with a particular focus on age-related diseases. These diseases, spanning cardiovascular and cerebrovascular disorders, malignancies, and neurodegenerative conditions, significantly contribute to the morbidity and mortality of older individuals. We delve into the fundamental cellular and molecular mechanisms underpinning aging, including mitochondrial dysfunction and cellular senescence, and elucidate their profound implications for the pathogenesis of various age-related diseases. Emphasis is placed on the importance of assessing key biomarkers of aging and biological age within the realm of pathology. We also scrutinize the interplay between cellular senescence and cancer biology as a central area of focus, underscoring its paramount significance in contemporary pathological research. Moreover, we shed light on the integration of anti-aging interventions that target fundamental aging processes, such as senolytics, mitochondria-targeted treatments, and interventions that influence epigenetic regulation within the domain of pathology research. In conclusion, the integration of geroscience concepts into pathological research heralds a transformative paradigm shift in our understanding of disease pathogenesis and promises breakthroughs in disease prevention and treatment.
Introduction
Geroscience, an emerging discipline at the intersection of aging and disease, seeks to understand the relationship between the aging process and pathogenesis of age-related diseases [1, 2]. Historically, the concept of cellular pathology laid the groundwork for modern pathology [3]. Today, geroscience promises to revolutionize this field by illuminating how aging mechanisms contribute to the pathogenesis of diseases associated with aging [4]. Geroscience has evolved significantly, fueled by discoveries that suggest that aging is governed by evolutionarily conserved cellular and molecular mechanisms and is a modifiable process. This perspective offers a novel lens through which the field of pathology can be viewed. Cellular aging is now viewed as a complex, regulated and potentially reversible process, governed by a genetic program, transcending the mere accumulation of macromolecular damage over time [4]. It is intricately intertwined with cellular dysfunction, playing a pivotal role in the onset of age-associated diseases. This perspective highlights that aging is not just a passive decline in cellular integrity but a coordinated series of events that might be amenable to targeted interventions.
Expanding on the core principles of geroscience, it’s important to delve into the fundamental, evolutionarily conserved aging mechanisms. These mechanisms, often referred to as the “pillars” or “hallmarks” of aging, drive the functional and phenotypic changes in aging cells [5]. Understanding these mechanisms is crucial for both geroscience and pathology, as they provide insights into the biological processes that underlie aging and age-related diseases. These mechanisms play a critical role in multitude of age-related diseases, including hematological diseases [6, 7], Alzheimer’s disease [8–12], osteoporosis, sarcopenia, various types of cancer, presbycusis and cardiovascular and cerebrovascular diseases [13–21]. In pathology, this link is crucial for understanding the etiology and progression of diseases that demonstrate an age-related increase in morbidity and mortality.
Hallmarks of aging and their pathological implications
Understanding the role of mechanisms/hallmarks of aging is pivotal in pathology, especially when examining age-related diseases [10, 22, 23]. Each of these aging mechanisms is intricately interconnected, collectively contributing to the pathology of a diverse array of age-related diseases (Figure 1). Their combined effects underscore the complexity of aging as a multi-faceted process, deeply influencing the onset and progression of various pathologies. Generally, a significant number of these mechanisms stem from spontaneous, stochastic damage, which in turn activates evolutionarily conserved cellular responses such as cellular senescence and inflammatory pathways. Concurrently, these processes are intertwined with pathways that determine cellular resilience to such damage or stress, including but not limited to the maintenance of proteostasis [24–28], the efficacy of Nrf2-driven antioxidant responses [29–36], and the integrity of DNA repair systems [37].
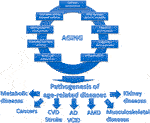
FIGURE 1. Interplay between hallmarks of aging and age-related diseases. This schematic figure illustrates the interplay between the fundamental cellular and molecular mechanisms of aging, collectively contributing to what we perceive as “aging.” The top section showcases these interconnected hallmarks of aging, encompassing processes such as cellular senescence, mitochondrial dysfunction, genomic instability, and heightened state of inflammation, among others. These synergistic aging processes promote the pathogenesis of a spectrum of diverse age-related diseases. These diseases, including but not limited to cardiovascular diseases (CVD), cancer, neurodegenerative disorders (including Alzheimer’s disease; AD), vascular cognitive impairment and dementia VCID), eye diseases (including age-related macular degeneration, AMD), musculoskeletal diseases and metabolic diseases, share a common origin in the aging process.
Recent advancements have revealed that cell-autonomous mechanisms alone do not fully account for the complexities of aging. Instead, aging of various organ systems is also governed by a hierarchical regulatory cascade, where cell-autonomous aging processes are modulated by systemic and circulating factors [28, 38–40]. Furthermore, age-related changes within cells often lead to non-cell autonomous effects, such as the release of paracrine mediators from senescent cells, which in turn influence tissue aging [41–43]. A unifying model suggests that the orchestration of aging processes across various organs involves a balance of circulating pro-geronic and anti-geronic factors. These factors, emanating from the central nervous system, endocrine organs, the immune system, adipose tissue, and even the gastrointestinal tract, play pivotal roles in orchestrating and harmonizing aging processes. An important implication of this concept is that conventional risk factors (including lifestyle [44–50] and environmental risk factors [51, 52]) exacerbate age-related pathologies by intensifying fundamental molecular and cellular aging processes, both cell autonomous and non-cell autonomous.
Since the free radical theory of aging was published in the 1950s, oxidative stress has been increasingly recognized as a key player in the aging process [53, 54]. While reactive oxygen species (ROS) play essential roles in cell signaling, excessive ROS production or inadequate ROS detoxification can lead to oxidative stress, damaging cells and tissues and causing cellular dysfunction [55–60]. In the context of aging, the balance between ROS production and antioxidant defenses becomes skewed. Age-related increases in oxidative stress are thought to contribute to cardiovascular pathologies [61–71], the deterioration of neurological function and development of brain and muscle aging phenotypes, among others. One of the most critical impacts of oxidative stress in aging is on cellular signaling pathways, including triggering of inflammatory mechanisms [30, 67].
The reduced capacity of older cells to counter molecular stress and achieve homeostasis is a critical aging phenomenon [72]. In youthful organisms, a rise in cellular reactive oxygen species prompts adaptive homeostatic responses, chiefly through the activation of the Nrf2 (Nuclear factor erythroid 2-related factor 2)-driven antioxidant defense pathway [30–32, 73]. Nrf2, a transcription factor sensitive to redox changes, coordinates this response, up-regulating the levels of enzymes that neutralize ROS and repair ROS-inflicted macromolecular damage [74]. This adaptive response effectively mitigates oxidative stress in younger cells. However, as organisms age, a significant reduction in Nrf2 functionality occurs, especially noticeable in vascular tissues. This reduction intensifies oxidative stress and increases the susceptibility of aged cells to ROS-related damage, potentially playing a pivotal role in the emergence of age-related pathologies [30, 31]. Notably, the beneficial anti-aging effects associated with caloric restriction are linked to the stimulation of these Nrf2-mediated pathways [29, 75].
Genomic instability, characterized by the accumulation of DNA damage from environmental stressors and metabolic activities, is a key contributor to aging [76]. This instability can result in mutations and chromosomal aberrations, which are central to the development of many age-related diseases, particularly cancer and cardiovascular diseases [18, 77, 78]. Recently, clonal hematopoiesis of indeterminate potential (CHIP) has emerged as a significant area of interest in geroscience [79–88]. CHIP refers to an age-related phenomenon where hematopoietic stem cells acquire somatic mutations that lead to pre-malignant clonal expansion of white blood cells [81–88]. These cells exhibit marked changes in their function, including a pro-inflammatory shift in their phenotype. Importantly, CHIP is not necessarily linked to hematological malignancies but is associated with an increased risk of developing a range of age-related diseases, including atherosclerosis [81–88].
Telomeres, which are protective caps at the ends of chromosomes, gradually shorten with each cell division [18, 89]. Excessive shortening contribute to cellular aging or apoptosis, impairing tissue repair and regeneration [90–93]. Telomere attrition is thought to contribute to many age-related diseases and conditions [18, 94–97].
Age-related changes in epigenetic regulation, such as DNA methylation [94, 98–103], affect gene expression and are implicated in dysregulation of various cellular processes with age and the pathogenesis of age-related diseases, including cancer [104], cardiovascular diseases [105] and Alzheimer’s disease.
With aging, the ability of cells to maintain protein homeostasis diminishes [106], leading to the accumulation of misfolded proteins [107]. Impaired protein homeostasis has been linked to the pathogenesis of a wide range of age-related diseases and conditions [108]. This loss is characteristic of neurodegenerative diseases like Parkinson’s disease and Alzheimer’s disease, where protein aggregates are a hallmark feature [109], disrupting normal organ function.
Aging affects nutrient-sensing pathways [110], impacting metabolism and cellular growth. Alterations in insulin signaling and mTOR pathways are considered evolutionarily conserved mechanisms of aging [111–113]. These changes are evident in age-related diseases like type 2 diabetes and are implicated in the pathogenesis of many age-related diseases, including various types of cancer [114–117] cardiovascular diseases and Alzheimer’s disease.
Autophagy, a multifaceted cellular pathway, stands as a central player in the intricate interplay between aging and disease processes [118–121]. It plays a pivotal role in maintaining cellular homeostasis by degrading and recycling damaged or dysfunctional cellular components, thereby promoting cellular renewal and survival. In the context of aging, autophagy’s efficacy tends to decline, leading to the accumulation of cellular debris and dysfunctional organelles, which are hallmarks of aging [122–125]. The influence of autophagy extends beyond cellular maintenance, as it significantly impacts immune regulation [126, 127] and tumorigenesis [128]. One of the critical regulatory connections involving autophagy is its interaction with the mTOR pathway [129, 130]. mTOR is a central regulator of cellular growth and metabolism, and it inhibits autophagy when nutrients are plentiful. Conversely, during nutrient scarcity or stress conditions, mTOR inhibition allows autophagy to proceed, promoting the removal of damaged cellular components and recycling of resources. This intricate balance between mTOR and autophagy is intimately tied to aging and disease [129, 130], as excessive mTOR activity and compromised autophagy are associated with age-related pathologies, including neurodegenerative diseases and cancer. In the context of immune regulation, autophagy plays a dual role [126, 127]. It contributes to the activation and functioning of immune cells, aiding in the presentation of antigens. Conversely, autophagy can also regulate immune responses, influencing immune tolerance and autoimmunity. Dysregulation of autophagy in immune cells can have profound consequences on an individual’s susceptibility to infections and autoimmune diseases [131]. Regarding tumorigenesis, autophagy’s role is complex [128]. It can promote tumor survival under certain conditions by providing cancer cells with nutrients during nutrient deprivation or stress. In summary, autophagy represents a multifaceted cellular pathway with far-reaching implications in aging. Its intricate relationship with mTOR highlights its significance in aging and disease processes, making it a compelling focus of research in the field of geroscience and cancer biology. Understanding how to modulate autophagy effectively may hold promise in promoting healthy aging and combating age-related diseases.
Sirtuins, a family of NAD+-dependent protein deacetylases, have emerged as pivotal regulators of cellular and organismal aging [132–140]. By modulating the acetylation status of a myriad of protein targets, Sirtuins regulate cellular metabolism, genomic stability, stress resilience and stress response pathways [141–150]. Through their influence on key cellular processes such as DNA repair, mitochondrial function, and inflammation, Sirtuins directly impact the rate of aging and age-related diseases [141–150]. Their intricate involvement in pathways related to calorie restriction and cellular homeostasis has led to substantial interest in their potential as therapeutic targets for extending healthspan and combating age-related diseases. Cytoplasmic (SIRT1, SIRT2, SIRT6) [151, 152], nuclear (SIRT1, SIRT2, SIRT6), nucleolar (SIRT7) [153], and mitochondrial (SIRT3, SIRT4, SIRT5) [154–158] sirtuins represent distinct subtypes within the broader family of sirtuins. SIRT1, the most extensively studied cytoplasmic sirtuin, is known for its specific roles in modulating cellular responses to nutrient availability and energy balance. It plays a crucial role in calorie restriction-related pathways, where it promotes the deacetylation and activation of various transcription factors, such as PGC-1α and FOXO, which are involved in metabolic processes, mitochondrial biogenesis, and antioxidant defense. SIRT2 regulates cytoskeletal dynamics and cell cycle progression, while SIRT6 and SIRT7 are implicated in DNA repair and chromatin maintenance, contributing to genomic stability. SIRT3, for example, promotes the deacetylation and activation of several mitochondrial proteins involved in oxidative phosphorylation and antioxidant defense, thereby enhancing mitochondrial efficiency and reducing oxidative stress. SIRT4 and SIRT5 play roles in regulating mitochondrial metabolism and amino acid metabolism, respectively. Dysregulation of cytoplasmic, nucleolar, or mitochondrial sirtuins can contribute to cellular dysfunction and accelerate the aging process, making them intriguing targets for interventions aimed at promoting healthy aging and mitigating age-related diseases.
Mitochondrial dysfunction is also a hallmark of aging [5, 159–161], impairing cellular energy metabolism and increasing oxidative stress, which profoundly affect cellular health and function. Mitochondrial dysfunction is thought to contribute to a range of age-related diseases [162], including neurodegenerative disorders [163], cardiovascular diseases [67, 72, 75, 164–166] and musculoskeletal diseases [167], including sarcopenia [168].
In aging dividing cells often enter a state of cellular senescence, impacting tissue function [169]. Cellular senescence is a DNA damage-induced cellular stress response, which is associated with profound changes in cellular phenotype [14]. Senescent cells secrete a range of inflammatory factors [170] and enzymes disrupting the extracellular matrix, contributing to tissue aging and dysfunction [171]. The accumulation of senescent cells is thought to be a driver of aging [111, 172–174] and have been linked to the pathogenesis of a number of age-related diseases [175], including musculoskeletal diseases [176, 177], neurodenegenenerative diseases [8, 10, 178] and VCI [179, 180], retina diseases [181] and cardiovascular diseases. The increased presence of senescent cells, often a result of DNA damage induced by lifestyle, environmental exposures [182], and certain interventions like anti-cancer therapies [183, 184], is believed to lead to accelerated aging, exacerbating the progression of age-related conditions and physiological decline. The intersection of cellular senescence biology and cancer biology represents a highly dynamic and increasingly pivotal area of interest in pathology, highlighting the complex relationship between aging processes and cancer development [185].
The regenerative potential of stem cells diminishes with age, affecting tissue repair and regeneration [186]. Stem cell exhaustion is thought to be an important factor in aging and age-related pathologies like musculoskeletal diseases and hematologic diseases.
Aging is associated with chronic low-grade sterile inflammation, often termed “inflammaging [171, 187–190],” which is a significant contributor to many age-related diseases, including cardiovascular diseases, neuroinflammation and neurodegenerative diseases [191–194], sarcopenia [195] and cognitive dysfunction [194, 196, 197].
In pathology, these cellular and molecular mechanisms provide a framework for understanding the complex interplay between aging and disease. By considering these mechanisms, pathologists can gain insights into the onset, progression, and potential therapeutic targets for a wide range of age-related conditions.
Anti-aging interventions and plasticity of aging
Anti-aging interventions, which specifically target one or more fundamental aging processes, hold immense potential in preventing the development of age-related diseases and altering their trajectories. These therapies, ranging from pharmacological [198–201] to dietary [202], nutraceutical [203], and lifestyle [204] interventions [205–207], aim to slow aging and prevent age-related pathologies. The burgeoning field of pharmacological anti-aging interventions is rapidly evolving, with several promising therapeutic avenues emerging. Among these, senolytics, which selectively target and eliminate senescent cells, are at the forefront [176, 180, 181, 208–210]. These agents offer potential in mitigating the detrimental effects of cellular senescence, a key contributor to age-related diseases and dysfunctions. Another area of significant translational relevance is mitochondria-targeted treatments [165, 211–213]. Treatments that modulate epigenetic regulation [214, 215] and restore cellular NAD levels [216–226] represent another exciting frontier. Additionally, targeting the mTOR pathway offers significant potential [12]. Inhibitors of the mTOR pathway have shown promising results in extending lifespan and healthspan in various model organisms [15, 113], suggesting their potential applicability in human aging.
As we move into the upcoming decade, we anticipate significant advancements in developing these novel interventions. The role of pathology in this progress is pivotal. With standardized assessment of key biomarkers of aging, pathology will play a crucial role in rigorously testing the efficacy of these interventions. This testing, critical both in preclinical studies and clinical trials, can include methodologies such as using tissue biopsies to assess the impact of these interventions on cellular and molecular levels. The integration of interventions that target cellular and molecular mechanisms of aging into pathological studies not only holds the potential for breakthroughs in disease prevention and treatment but also promises to revolutionize our understanding of disease pathogenesis. This revolution involves introducing geroscience concepts into pathology, further bridging the gap between aging research and clinical practice.
Novel tools and experimental models from geroscience
Geroscience has developed an array of tools and models over the past decade that can be adapted to pathology research. One key area is the use of aging models to study diseases. Traditionally, research on age-related diseases like cancer, stroke, hypertension, and myocardial infarction has been regularly conducted using young animal models. However, these models often fail to capture the complexities of human diseases, which typically manifest in older populations. By employing aging models, pathologists can better understand how these diseases develop and progress in an aging body, leading to more accurate representations and insights.
The shift to age-relevant disease models
The integration of age-appropriate models in pathological studies is crucial. Diseases in older adults often present differently than in younger individuals, both in terms of symptoms, severity and underlying pathophysiology. By using preclinical models that more closely resemble the chronological age at which these diseases commonly occur in humans, researchers can uncover new aspects of disease mechanisms that were previously obscured in younger models. This shift could lead to the development of more effective diagnostic tools and treatments that are tailored to the older population actually affected by these diseases.
The COVID-19 pandemic has starkly highlighted the importance of age-relevant disease models in pathology [227, 228]. COVID-19’s associated pathologies extend beyond respiratory symptoms, encompassing a range of complications including lung injury, systemic microvascular damage, cardiac abnormalities, and neurological manifestations [229–239]. The consequences of infection with SARS-CoV-2 have been particularly severe in older adults [240, 241] and in individuals with accelerated biological aging due to factors such as obesity [242–254]. Geroscience has been instrumental in uncovering how specific age-related mechanisms may contribute to this increased disease severity [244–246, 248, 249, 252, 255, 256]. For example, aging-related changes in the immune system, known as immunosenescence, and inflammaging, are believed to play key roles in the heightened vulnerability and severity of COVID-19 in the older population [257–262]. Understanding the unique impact of COVID-19 on older adults is essential, making the use of age-relevant models in research crucial.
Utilizing models targeting aging mechanisms in pathology
Pathology stands to gain substantially from integrating models that target aging mechanisms, a key area of focus in geroscience research. Cellular senescence serves as a prime example of an aging process with profound implications for disease development and progression. Over the past decade, a variety of murine models have been developed, allowing for the precise detection and elimination of senescent cells [183, 263–266]. This advancement has shed light on the role of senescent cells in the pathogenesis of numerous age-related diseases. By adopting these geroscience-derived models, pathologists can broaden their investigative scope, targeting a wider array of diseases. This approach not only deepens our understanding of the cellular and molecular foundations of these conditions but also paves the way for more targeted diagnostic and therapeutic strategies in a range of age-related diseases. Fields such as cancer research [263] and musculoskeletal [265–267], ophthalmological [268, 269], otological, and hematological [6, 264] research in pathology are prime examples where an interdisciplinary approach, integrating a geroscience perspective, could lead to significant breakthroughs. By embracing this cross-disciplinary methodology, pathologists can uncover novel insights and develop groundbreaking treatments in these specialized areas, all deeply influenced by the aging process.
Epigenetic clocks and biological age assessment
Methods developed to assess mechanisms of aging and biological age [100, 270–275], such as epigenetic clocks [47, 94, 100–102, 276–281], offer valuable tools for pathological studies. These methods can be used to determine the biological age of tissues and cells, which may differ from chronological age. Incorporating these measurements into pathological research can provide new insights into the relationship between aging and disease, and potentially uncover new biomarkers for early detection and intervention.
Conclusion
In summary, geroscience offers a transformative perspective for pathology, bridging the gap between aging processes and disease pathogenesis. By adopting age-appropriate disease models and preclinical models that target specific aging mechanisms, utilizing tools to assess biological aging, and embracing an interdisciplinary approach, pathology can greatly enhance its understanding and treatment of age-related diseases. This integration marks a pivotal shift in the approach to disease research, offering the promise of effective and targeted interventions for the aging population. As the field of geroscience continues to evolve, it will undoubtedly become an integral part of pathology, offering new insights and diagnostic protocols.
The National Institute on Aging (NIA) in the United States has established a vital network of core facilities known as the Nathan Shock Centers of Excellence in the Biology of Aging [282–290]. These centers offer specialized services on a fee-for-service basis to researchers aiming to characterize specific mechanisms of aging and assess biological age. Such initiatives play a crucial role in fostering the incorporation of geroscience concepts into pathological research. By providing access to advanced technologies and expertise, these centers enable researchers to delve deeper into the complexities of aging at a molecular and cellular level [282–290]. This approach not only enhances our understanding of the aging process but also accelerates the development of targeted interventions for age-related diseases. The success of the Nathan Shock Centers serves as a model that could be replicated by other countries, particularly in the European Union, to promote global collaboration and advancement in aging research. By establishing similar facilities, there can be a concerted effort towards integrating geroscience more broadly into various fields of study, including pathology, thereby paving the way for groundbreaking discoveries and innovations in the science of aging and pathology and pathophysiology of age-related diseases.
Author contributions
AL conceptualized the article. AL wrote the first draft. MF, VF-P, DM, and AF all revised the manuscript critically. All authors contributed to the article and approved the submitted version.
Funding
Project no. TKP2021-NKTA-47, implemented with the support provided by the Ministry of Innovation and Technology of Hungary from the National Research, Development and Innovation Fund, financed under the TKP2021-NKTA funding scheme; by funding through the National Cardiovascular Laboratory Program (RRF-2.3.1-21-2022-00003) provided by the Ministry of Innovation and Technology of Hungary from the National Research, Development and Innovation Fund; Project no. 135784 implemented with the support provided from the National Research, Development and Innovation Fund of Hungary, financed under the K_20 funding scheme and the European University for Wellbeing (EUniWell) program (grant agreement number: 101004093/EUniWell/EAC-A02-2019/EAC-A02-2019-1). The funding sources had no role in the writing of the manuscript; and in the decision to submit the article for publication. The 3.5 version of ChatGPT, developed by OpenAI, was used as a tool to refine our writing and enhancing the clarity of our work.
Conflict of interest
The authors declare that the research was conducted in the absence of any commercial or financial relationships that could be construed as a potential conflict of interest.
References
1. Hernandez, CM, Hernandez, AR, Hoffman, JM, King, PH, McMahon, LL, Buford, TW, et al. A neuroscience primer for integrating geroscience with the neurobiology of aging. J Gerontol A Biol Sci Med Sci (2022) 77:e19–e33. doi:10.1093/gerona/glab301
2. Llarena, N, and Hine, C. Reproductive longevity and aging: geroscience approaches to maintain long-term ovarian fitness. J Gerontol A Biol Sci Med Sci (2021) 76:1551–60. doi:10.1093/gerona/glaa204
4. Duque, G, Lipsitz, LA, Ferrucci, L, Addie, S, Carrington-Lawrence, S, and Kohanski, R. Geroscience for the next chapter of medicine. J Gerontol A Biol Sci Med Sci (2023) 78:791–2. doi:10.1093/gerona/glad083
5. Lopez-Otin, C, Blasco, MA, Partridge, L, Serrano, M, and Kroemer, G. The hallmarks of aging. Cell (2013) 153:1194–217. doi:10.1016/j.cell.2013.05.039
6. Urban, VS, Cegledi, A, and Mikala, G. Multiple myeloma, a quintessential malignant disease of aging: a geroscience perspective on pathogenesis and treatment. Geroscience (2023) 45:727–46. doi:10.1007/s11357-022-00698-x
7. Lamanuzzi, A, Saltarella, I, Frassanito, MA, Ribatti, D, Melaccio, A, Desantis, V, et al. Thrombopoietin promotes angiogenesis and disease progression in patients with multiple myeloma. Am J Pathol (2021) 191:748–58. doi:10.1016/j.ajpath.2020.12.016
8. Dorigatti, AO, Riordan, R, Yu, Z, Ross, G, Wang, R, Reynolds-Lallement, N, et al. Brain cellular senescence in mouse models of Alzheimer's disease. Geroscience (2022) 44:1157–68. doi:10.1007/s11357-022-00531-5
9. Pomilio, C, Gorojod, RM, Riudavets, M, Vinuesa, A, Presa, J, Gregosa, A, et al. Microglial autophagy is impaired by prolonged exposure to β-amyloid peptides: evidence from experimental models and Alzheimer's disease patients. Geroscience (2020) 42:613–32. doi:10.1007/s11357-020-00161-9
10. DiBattista, AM, Sierra, F, and Masliah, E. NIA workshop on senescence in brain aging and Alzheimer's disease and its related dementias. Geroscience (2020) 42:389–96. doi:10.1007/s11357-020-00153-9
11. Liu, H, Lutz, M, and Luo, S, Alzheimer’s Disease Neuroimaging Initiative. Genetic association between epigenetic aging-acceleration and the progression of mild cognitive impairment to Alzheimer's disease. J Gerontol A Biol Sci Med Sci (2022) 77:1734–42. doi:10.1093/gerona/glac138
12. Wang, H, Fu, J, Xu, X, Yang, Z, and Zhang, T. Rapamycin activates mitophagy and alleviates cognitive and synaptic plasticity deficits in a mouse model of Alzheimer's disease. J Gerontol A Biol Sci Med Sci (2021) 76:1707–13. doi:10.1093/gerona/glab142
13. Toth, L, Czigler, A, Hegedus, E, Komaromy, H, Amrein, K, Czeiter, E, et al. Age-related decline in circulating IGF-1 associates with impaired neurovascular coupling responses in older adults. Geroscience (2022) 44:2771–83. doi:10.1007/s11357-022-00623-2
14. Sakamuri, S, Sure, VN, Kolli, L, Liu, N, Evans, WR, Sperling, JA, et al. Glycolytic and oxidative phosphorylation defects precede the development of senescence in primary human brain microvascular endothelial cells. Geroscience (2022) 44:1975–94. doi:10.1007/s11357-022-00550-2
15. Towner, RA, Gulej, R, Zalles, M, Saunders, D, Smith, N, Lerner, M, et al. Rapamycin restores brain vasculature, metabolism, and blood-brain barrier in an inflammaging model. Geroscience (2021) 43:563–78. doi:10.1007/s11357-021-00363-9
16. Islam, MT, Tuday, E, Allen, S, Kim, J, Trott, DW, Holland, WL, et al. Senolytic drugs, dasatinib and quercetin, attenuate adipose tissue inflammation, and ameliorate metabolic function in old age. Aging Cell (2023) 22:e13767. doi:10.1111/acel.13767
17. Bloom, SI, Tucker, JR, Machin, DR, Abdeahad, H, Adeyemo, AO, Thomas, TG, et al. Reduction of double-strand DNA break repair exacerbates vascular aging. Aging (Albany NY) (2023) 15:9913–47. doi:10.18632/aging.205066
18. Bloom, SI, Tucker, JR, Lim, J, Thomas, TG, Stoddard, GJ, Lesniewski, LA, et al. Aging results in DNA damage and telomere dysfunction that is greater in endothelial versus vascular smooth muscle cells and is exacerbated in atheroprone regions. Geroscience (2022) 44:2741–55. doi:10.1007/s11357-022-00681-6
19. Ungvari, Z, Tarantini, S, Donato, AJ, Galvan, V, and Csiszar, A. Mechanisms of vascular aging. Circ Res (2018) 123:849–67. doi:10.1161/CIRCRESAHA.118.311378
20. Liu, Y, Bloom, SI, and Donato, AJ. The role of senescence, telomere dysfunction and shelterin in vascular aging. Microcirculation (2018) 26:e12487. doi:10.1111/micc.12487
21. Rossman, MJ, Kaplon, RE, Hill, SD, McNamara, MN, Santos-Parker, JR, Pierce, GL, et al. Endothelial cell senescence with aging in healthy humans: prevention by habitual exercise and relation to vascular endothelial function. Am J Physiol Heart Circ Physiol (2017) 313:H890–H895. doi:10.1152/ajpheart.00416.2017
22. Kennedy, BK, Berger, SL, Brunet, A, Campisi, J, Cuervo, AM, Epel, ES, et al. Geroscience: linking aging to chronic disease. Cell (2014) 159:709–13. doi:10.1016/j.cell.2014.10.039
23. Rolland, Y, Sierra, F, Ferrucci, L, Barzilai, N, De Cabo, R, Mannick, J, et al. Challenges in developing Geroscience trials. Nat Commun (2023) 14:5038. doi:10.1038/s41467-023-39786-7
24. Kaushik, S, and Cuervo, AM. Proteostasis and aging. Nat Med (2015) 21:1406–15. doi:10.1038/nm.4001
25. Korovila, I, Hugo, M, Castro, JP, Weber, D, Hohn, A, Grune, T, et al. Proteostasis, oxidative stress and aging. Redox Biol (2017) 13:550–67. doi:10.1016/j.redox.2017.07.008
26. Santra, M, Dill, KA, and de Graff, AMR. Proteostasis collapse is a driver of cell aging and death. Proc Natl Acad Sci U S A (2019) 116:22173–8. doi:10.1073/pnas.1906592116
27. Taylor, RC, and Hetz, C. Mastering organismal aging through the endoplasmic reticulum proteostasis network. Aging Cell (2020) 19:e13265. doi:10.1111/acel.13265
28. Ferreira, JV, da Rosa Soares, A, and Pereira, P. Cell non-autonomous proteostasis regulation in aging and disease. Front Neurosci (2022) 16:878296. doi:10.3389/fnins.2022.878296
29. Pearson, KJ, Lewis, KN, Price, NL, Chang, JW, Perez, E, Cascajo, MV, et al. Nrf2 mediates cancer protection but not prolongevity induced by caloric restriction. Proc Natl Acad Sci U S A (2008) 105:2325–30. doi:10.1073/pnas.0712162105
30. Ungvari, Z, Bailey-Downs, L, Gautam, T, Sosnowska, D, Wang, M, Monticone, RE, et al. Age-associated vascular oxidative stress, Nrf2 dysfunction, and NF-{kappa}B activation in the nonhuman primate Macaca mulatta. J Gerontol A Biol Sci Med Sci (2011) 66:866–75. doi:10.1093/gerona/glr092
31. Ungvari, Z, Bailey-Downs, L, Sosnowska, D, Gautam, T, Koncz, P, Losonczy, G, et al. Vascular oxidative stress in aging: a homeostatic failure due to dysregulation of Nrf2-mediated antioxidant response. Am J Physiol Heart Circ Physiol (2011) 301:H363–72. doi:10.1152/ajpheart.01134.2010
32. Ungvari, ZI, Bailey-Downs, L, Gautam, T, Jimenez, R, Losonczy, G, Zhang, C, et al. Adaptive induction of NF-E2-Related Factor-2-driven antioxidant genes in endothelial cells in response to hyperglycemia. Am J Physiol Heart Circ Physiol (2011) 300:H1133–40. doi:10.1152/ajpheart.00402.2010
33. Ahn, B, Pharaoh, G, Premkumar, P, Huseman, K, Ranjit, R, Kinter, M, et al. Nrf2 deficiency exacerbates age-related contractile dysfunction and loss of skeletal muscle mass. Redox Biol (2018) 17:47–58. doi:10.1016/j.redox.2018.04.004
34. Fulop, GA, Kiss, T, Tarantini, S, Balasubramanian, P, Yabluchanskiy, A, Farkas, E, et al. Nrf2 deficiency in aged mice exacerbates cellular senescence promoting cerebrovascular inflammation. Geroscience (2018) 40:513–21. doi:10.1007/s11357-018-0047-6
35. Tarantini, S, Valcarcel-Ares, MN, Yabluchanskiy, A, Tucsek, Z, Hertelendy, P, Kiss, T, et al. Nrf2 deficiency exacerbates obesity-induced oxidative stress, neurovascular dysfunction, blood brain barrier disruption, neuroinflammation, amyloidogenic gene expression and cognitive decline in mice, mimicking the aging phenotype. J Gerontol A Biol Sci Med Sci (2018) 73:853–63. doi:10.1093/gerona/glx177
36. Ungvari, Z, Tarantini, S, Nyul-Toth, A, Kiss, T, Yabluchanskiy, A, Csipo, T, et al. Nrf2 dysfunction and impaired cellular resilience to oxidative stressors in the aged vasculature: from increased cellular senescence to the pathogenesis of age-related vascular diseases. Geroscience (2019) 41:727–38. doi:10.1007/s11357-019-00107-w
37. Maynard, S, Fang, EF, Scheibye-Knudsen, M, Croteau, DL, and Bohr, VA. DNA damage, DNA repair, aging, and neurodegeneration. Cold Spring Harb Perspect Med (2015) 5:a025130. doi:10.1101/cshperspect.a025130
38. Yousefzadeh, MJ, Wilkinson, JE, Hughes, B, Gadela, N, Ladiges, WC, Vo, N, et al. Heterochronic parabiosis regulates the extent of cellular senescence in multiple tissues. Geroscience (2020) 42:951–61. doi:10.1007/s11357-020-00185-1
39. Gonzalez-Armenta, JL, Li, N, Lee, RL, Lu, B, and Molina, AJA. Heterochronic parabiosis: old blood induces changes in mitochondrial structure and function of young mice. J Gerontol A Biol Sci Med Sci (2021) 76:434–9. doi:10.1093/gerona/glaa299
40. Miller, HA, Dean, ES, Pletcher, SD, and Leiser, SF. Cell non-autonomous regulation of health and longevity. Elife (2020) 9:e62659. doi:10.7554/eLife.62659
41. Lecot, P, Alimirah, F, Desprez, PY, Campisi, J, and Wiley, C. Context-dependent effects of cellular senescence in cancer development. Br J Cancer (2016) 114:1180–4. doi:10.1038/bjc.2016.115
42. Chinta, SJ, Woods, G, Rane, A, Demaria, M, Campisi, J, and Andersen, JK. Cellular senescence and the aging brain. Exp Gerontol (2014) 68:3–7. doi:10.1016/j.exger.2014.09.018
43. Tchkonia, T, Zhu, Y, van Deursen, J, Campisi, J, and Kirkland, JL. Cellular senescence and the senescent secretory phenotype: therapeutic opportunities. J Clin Invest (2013) 123:966–72. doi:10.1172/JCI64098
44. Henderson, YO, Bithi, N, Yang, J, Link, C, Zhang, A, Baron, B, et al. A long-term obesogenic high-fat diet in mice partially dampens the anti-frailty benefits of late-life intermittent fasting. Geroscience (2023) 45:1247–62. doi:10.1007/s11357-022-00678-1
45. Nunan, E, Wright, CL, Semola, OA, Subramanian, M, Balasubramanian, P, Lovern, PC, et al. Obesity as a premature aging phenotype - implications for sarcopenic obesity. Geroscience (2022) 44:1393–405. doi:10.1007/s11357-022-00567-7
46. Seligman, BJ, Berry, SD, Lipsitz, LA, Travison, TG, and Kiel, DP. Epigenetic age acceleration and change in frailty in MOBILIZE boston. J Gerontol A Biol Sci Med Sci (2022) 77:1760–5. doi:10.1093/gerona/glac019
47. O'Shea, DM, Maynard, T, and Tremont, G. DNA methylation "GrimAge" acceleration mediates sex/gender differences in verbal memory and processing speed: findings from the health and retirement study. J Gerontol A Biol Sci Med Sci (2022) 77:2402–12. doi:10.1093/gerona/glac133
48. Lin, WY. Lifestyle factors and genetic variants on 2 biological age measures: evidence from 94 443 taiwan biobank participants. J Gerontol A Biol Sci Med Sci (2022) 77:1189–98. doi:10.1093/gerona/glab251
49. Zhou, YF, Song, XY, Pan, XF, Feng, L, Luo, N, Yuan, JM, et al. Association between combined lifestyle factors and healthy ageing in Chinese adults: the Singapore Chinese health study. J Gerontol A Biol Sci Med Sci (2021) 76:1796–805. doi:10.1093/gerona/glab033
50. Wu, Q, Ailshire, JA, Kim, JK, and Crimmins, EM. Cardiometabolic risk trajectory among older Americans: findings from the health and retirement study. J Gerontol A Biol Sci Med Sci (2021) 76:2265–74. doi:10.1093/gerona/glab205
51. Pandics, T, Major, D, Fazekas-Pongor, V, Szarvas, Z, Peterfi, A, Mukli, P, et al. Exposome and unhealthy aging: environmental drivers from air pollution to occupational exposures. Geroscience (2023) 45:3381–408. doi:10.1007/s11357-023-00913-3
52. Chen, GC, Hukportie, DN, Zhongxiao, W, Li, FR, and Wu, XB. The association between exposure to air pollution and dementia incidence: the modifying effect of smoking. J Gerontol A Biol Sci Med Sci (2022) 78:2309–17. doi:10.1093/gerona/glac228
53. Harman, D. Aging: a theory based on free radical and radiation chemistry. J Gerontol (1956) 11:298–300. doi:10.1093/geronj/11.3.298
54. Harman, D. The biologic clock: the mitochondria? J Am Geriatr Soc (1972) 20:145–7. doi:10.1111/j.1532-5415.1972.tb00787.x
55. Oudbier, SJ, Goh, J, Looijaard, S, Reijnierse, EM, Meskers, CGM, and Maier, AB. Pathophysiological mechanisms explaining the association between low skeletal muscle mass and cognitive function. J Gerontol A Biol Sci Med Sci (2022) 77:1959–68. doi:10.1093/gerona/glac121
56. Liao, FF, Lin, G, Chen, X, Chen, L, Zheng, W, Raghow, R, et al. Endothelial nitric oxide synthase-deficient mice: a model of spontaneous cerebral small-vessel disease. Am J Pathol (2021) 191:1932–45. doi:10.1016/j.ajpath.2021.02.022
57. Serikbaeva, A, Li, Y, Ganesh, B, Zelkha, R, and Kazlauskas, A. Hyperglycemia promotes mitophagy and thereby mitigates hyperglycemia-induced damage. Am J Pathol (2022) 192:1779–94. doi:10.1016/j.ajpath.2022.08.004
58. Ionescu-Tucker, A, and Cotman, CW. Emerging roles of oxidative stress in brain aging and Alzheimer's disease. Neurobiol Aging (2021) 107:86–95. doi:10.1016/j.neurobiolaging.2021.07.014
59. Logan, S, Royce, GH, Owen, D, Farley, J, Ranjo-Bishop, M, Sonntag, WE, et al. Accelerated decline in cognition in a mouse model of increased oxidative stress. Geroscience (2019) 41:591–607. doi:10.1007/s11357-019-00105-y
60. Dai, DF, Chiao, YA, Marcinek, DJ, Szeto, HH, and Rabinovitch, PS. Mitochondrial oxidative stress in aging and healthspan. Longev Healthspan (2014) 3:6. doi:10.1186/2046-2395-3-6
61. Csiszar, A, Ungvari, Z, Edwards, JG, Kaminski, PM, Wolin, MS, Koller, A, et al. Aging-induced phenotypic changes and oxidative stress impair coronary arteriolar function. Circ Res (2002) 90:1159–66. doi:10.1161/01.res.0000020401.61826.ea
62. Sun, D, Huang, A, Yan, EH, Wu, Z, Yan, C, Kaminski, PM, et al. Reduced release of nitric oxide to shear stress in mesenteric arteries of aged rats. Am J Physiol Heart Circ Physiol (2004) 286:H2249–56. doi:10.1152/ajpheart.00854.2003
63. Hamilton, CA, Brosnan, MJ, McIntyre, M, Graham, D, and Dominiczak, AF. Superoxide excess in hypertension and aging: a common cause of endothelial dysfunction. Hypertension (2001) 37:529–34. doi:10.1161/01.hyp.37.2.529
64. van der Loo, B, Labugger, R, Skepper, JN, Bachschmid, M, Kilo, J, Powell, JM, et al. Enhanced peroxynitrite formation is associated with vascular aging. J Exp Med (2000) 192:1731–44. doi:10.1084/jem.192.12.1731
65. Francia, P, delli Gatti, C, Bachschmid, M, Martin-Padura, I, Savoia, C, Migliaccio, E, et al. Deletion of p66shc gene protects against age-related endothelial dysfunction. Circulation (2004) 110:2889–95. doi:10.1161/01.CIR.0000147731.24444.4D
66. Csiszar, A, Labinskyy, N, Orosz, Z, Xiangmin, Z, Buffenstein, R, and Ungvari, Z. Vascular aging in the longest-living rodent, the naked mole-rat. Am J Physiol (2007) 293:H919–27. doi:10.1152/ajpheart.01287.2006
67. Ungvari, ZI, Orosz, Z, Labinskyy, N, Rivera, A, Xiangmin, Z, Smith, KE, et al. Increased mitochondrial H2O2 production promotes endothelial NF-kappaB activation in aged rat arteries. Am J Physiol Heart Circ Physiol (2007) 293:H37–47. doi:10.1152/ajpheart.01346.2006
68. Jablonski, KL, Seals, DR, Eskurza, I, Monahan, KD, and Donato, AJ. High-dose ascorbic acid infusion abolishes chronic vasoconstriction and restores resting leg blood flow in healthy older men. J Appl Physiol (2007) 103:1715–21. doi:10.1152/japplphysiol.00533.2007
69. Donato, AJ, Eskurza, I, Silver, AE, Levy, AS, Pierce, GL, Gates, PE, et al. Direct evidence of endothelial oxidative stress with aging in humans: relation to impaired endothelium-dependent dilation and upregulation of nuclear factor-kappaB. Circ Res (2007) 100:1659–66. doi:10.1161/01.RES.0000269183.13937.e8
70. Fleenor, BS, Eng, JS, Sindler, AL, Pham, BT, Kloor, JD, and Seals, DR. Superoxide signaling in perivascular adipose tissue promotes age-related artery stiffness. Aging Cell (2014) 13:576–8. doi:10.1111/acel.12196
71. Toth, P, Tarantini, S, Springo, Z, Tucsek, Z, Gautam, T, Giles, CB, et al. Aging exacerbates hypertension-induced cerebral microhemorrhages in mice: role of resveratrol treatment in vasoprotection. Aging Cell (2015) 14:400–8. doi:10.1111/acel.12315
72. Ashton, KJ, Kiessling, CJ, Thompson, JM, Aziz, AY, Thomas, WG, Headrick, JP, et al. Early cardiac aging linked to impaired stress-resistance and transcriptional control of stress response, quality control and mitochondrial pathways. Exp Gerontol (2023) 171:112011. doi:10.1016/j.exger.2022.112011
73. de Souza, RG, Yu, Z, Hernandez, H, Trujillo-Vargas, CM, Lee, A, Mauk, KE, et al. Modulation of oxidative stress and inflammation in the aged lacrimal gland. Am J Pathol (2021) 191:294–308. doi:10.1016/j.ajpath.2020.10.013
74. Suh, JH, Shenvi, SV, Dixon, BM, Liu, H, Jaiswal, AK, Liu, RM, et al. Decline in transcriptional activity of Nrf2 causes age-related loss of glutathione synthesis, which is reversible with lipoic acid. Proc Natl Acad Sci U S A (2004) 101:3381–6. doi:10.1073/pnas.0400282101
75. Csiszar, A, Gautam, T, Sosnowska, D, Tarantini, S, Banki, E, Tucsek, Z, et al. Caloric restriction confers persistent anti-oxidative, pro-angiogenic, and anti-inflammatory effects and promotes anti-aging miRNA expression profile in cerebromicrovascular endothelial cells of aged rats. Am J Physiol Heart Circ Physiol (2014) 307:H292–306. doi:10.1152/ajpheart.00307.2014
76. Martin, GM, Hisama, FM, and Oshima, J. Review of how genetic research on segmental progeroid syndromes has documented genomic instability as a hallmark of aging but let us now pursue antigeroid syndromes. J Gerontol A Biol Sci Med Sci (2021) 76:253–9. doi:10.1093/gerona/glaa273
77. Durik, M, Kavousi, M, van der Pluijm, I, Isaacs, A, Cheng, C, Verdonk, K, et al. Nucleotide excision DNA repair is associated with age-related vascular dysfunction. Circulation (2012) 126:468–78. doi:10.1161/CIRCULATIONAHA.112.104380
78. Gray, K, Kumar, S, Figg, N, Harrison, J, Baker, L, Mercer, J, et al. Effects of DNA damage in smooth muscle cells in atherosclerosis. Circ Res (2015) 116:816–26. doi:10.1161/CIRCRESAHA.116.304921
79. Diez-Diez, M, Amoros-Perez, M, de la Barrera, J, Vazquez, E, Quintas, A, Pascual-Figal, DA, et al. Clonal hematopoiesis is not prevalent in Hutchinson-Gilford progeria syndrome. Geroscience (2023) 45:1231–6. doi:10.1007/s11357-022-00607-2
80. Del Pozo-Valero, M, Corton, M, Lopez-Rodriguez, R, Mahillo-Fernandez, I, Ruiz-Hornillos, J, Minguez, P, et al. Age-dependent association of clonal hematopoiesis with COVID-19 mortality in patients over 60 years. Geroscience (2023) 45:543–53. doi:10.1007/s11357-022-00666-5
81. Steensma, DP, Bejar, R, Jaiswal, S, Lindsley, RC, Sekeres, MA, Hasserjian, RP, et al. Clonal hematopoiesis of indeterminate potential and its distinction from myelodysplastic syndromes. Blood (2015) 126:9–16. doi:10.1182/blood-2015-03-631747
82. Jaiswal, S, Natarajan, P, Silver, AJ, Gibson, CJ, Bick, AG, Shvartz, E, et al. Clonal hematopoiesis and risk of atherosclerotic cardiovascular disease. N Engl J Med (2017) 377:111–21. doi:10.1056/NEJMoa1701719
83. Fuster, JJ, and Walsh, K. Somatic mutations and clonal hematopoiesis: unexpected potential new drivers of age-related cardiovascular disease. Circ Res (2018) 122:523–32. doi:10.1161/CIRCRESAHA.117.312115
84. Libby, P, and Ebert, BL. CHIP (clonal hematopoiesis of indeterminate potential): potent and newly recognized contributor to cardiovascular risk. Circulation (2018) 138:666–8. doi:10.1161/CIRCULATIONAHA.118.034392
85. Khetarpal, SA, Qamar, A, Bick, AG, Fuster, JJ, Kathiresan, S, Jaiswal, S, et al. Clonal hematopoiesis of indeterminate potential reshapes age-related CVD: JACC review topic of the week. J Am Coll Cardiol (2019) 74:578–86. doi:10.1016/j.jacc.2019.05.045
86. Nachun, D, Lu, AT, Bick, AG, Natarajan, P, Weinstock, J, Szeto, MD, et al. Clonal hematopoiesis associated with epigenetic aging and clinical outcomes. Aging Cell (2021) 20:e13366. doi:10.1111/acel.13366
87. Bhattacharya, R, Zekavat, SM, Haessler, J, Fornage, M, Raffield, L, Uddin, MM, et al. Clonal hematopoiesis is associated with higher risk of stroke. Stroke (2022) 53:788–97. doi:10.1161/STROKEAHA.121.037388
88. Uddin, MDM, Nguyen, NQH, Yu, B, Brody, JA, Pampana, A, Nakao, T, et al. Clonal hematopoiesis of indeterminate potential, DNA methylation, and risk for coronary artery disease. Nat Commun (2022) 13:5350. doi:10.1038/s41467-022-33093-3
89. Huang, Z, Liu, C, Ruan, Y, Guo, Y, Sun, S, Shi, Y, et al. Dynamics of leukocyte telomere length in adults aged 50 and older: a longitudinal population-based cohort study. Geroscience (2021) 43:645–54. doi:10.1007/s11357-020-00320-y
90. Sindi, S, Solomon, A, Kareholt, I, Hovatta, I, Antikainen, R, Hanninen, T, et al. Telomere length change in a multidomain lifestyle intervention to prevent cognitive decline: a randomized clinical trial. J Gerontol A Biol Sci Med Sci (2021) 76:491–8. doi:10.1093/gerona/glaa279
91. Pudas, S, Josefsson, M, Nordin Adolfsson, A, Landfors, M, Kauppi, K, Veng-Taasti, LM, et al. Short leukocyte telomeres, but not telomere attrition rates, predict memory decline in the 20-year longitudinal betula study. J Gerontol A Biol Sci Med Sci (2021) 76:955–63. doi:10.1093/gerona/glaa322
92. Kemp, BR, and Ferraro, KF. Are biological consequences of childhood exposures detectable in telomere length decades later? J Gerontol A Biol Sci Med Sci (2021) 76:7–14. doi:10.1093/gerona/glaa019
93. Demanelis, K, Tong, L, and Pierce, BL. Genetically increased telomere length and aging-related traits in the U.K. Biobank. J Gerontol A Biol Sci Med Sci (2021) 76:15–22. doi:10.1093/gerona/glz240
94. Pearce, EE, Alsaggaf, R, Katta, S, Dagnall, C, Aubert, G, Hicks, BD, et al. Telomere length and epigenetic clocks as markers of cellular aging: a comparative study. Geroscience (2022) 44:1861–9. doi:10.1007/s11357-022-00586-4
95. Zhan, Y, and Hagg, S. Association between genetically predicted telomere length and facial skin aging in the UK Biobank: a Mendelian randomization study. Geroscience (2021) 43:1519–25. doi:10.1007/s11357-020-00283-0
96. El Assar, M, Angulo, J, Carnicero, JA, Walter, S, Garcia-Garcia, FJ, Rodriguez-Artalejo, F, et al. Association between telomere length, frailty and death in older adults. Geroscience (2021) 43:1015–27. doi:10.1007/s11357-020-00291-0
97. Katz, R, Gay, EL, Kuipers, AL, Lee, JH, Honig, LS, Christensen, K, et al. Association of leukocyte telomere length with perceived physical fatigability. Exp Gerontol (2022) 170:111988. doi:10.1016/j.exger.2022.111988
98. Yusipov, I, Kondakova, E, Kalyakulina, A, Krivonosov, M, Lobanova, N, Bacalini, MG, et al. Accelerated epigenetic aging and inflammatory/immunological profile (ipAGE) in patients with chronic kidney disease. Geroscience (2022) 44:817–34. doi:10.1007/s11357-022-00540-4
99. Vetter, VM, Sommerer, Y, Kalies, CH, Spira, D, Bertram, L, and Demuth, I. Vitamin D supplementation is associated with slower epigenetic aging. Geroscience (2022) 44:1847–59. doi:10.1007/s11357-022-00581-9
100. Fraszczyk, E, Thio, CHL, Wackers, P, Dolle, MET, Bloks, VW, Hodemaekers, H, et al. DNA methylation trajectories and accelerated epigenetic aging in incident type 2 diabetes. Geroscience (2022) 44:2671–84. doi:10.1007/s11357-022-00626-z
101. Schachtschneider, KM, Schook, LB, Meudt, JJ, Shanmuganayagam, D, Zoller, JA, Haghani, A, et al. Epigenetic clock and DNA methylation analysis of porcine models of aging and obesity. Geroscience (2021) 43:2467–83. doi:10.1007/s11357-021-00439-6
102. Horvath, S, Zoller, JA, Haghani, A, Lu, AT, Raj, K, Jasinska, AJ, et al. DNA methylation age analysis of rapamycin in common marmosets. Geroscience (2021) 43:2413–25. doi:10.1007/s11357-021-00438-7
103. Horvath, S, Zoller, JA, Haghani, A, Jasinska, AJ, Raj, K, Breeze, CE, et al. Epigenetic clock and methylation studies in the rhesus macaque. Geroscience (2021) 43:2441–53. doi:10.1007/s11357-021-00429-8
104. Tolkach, Y, Zarbl, R, Bauer, S, Ritter, M, Ellinger, J, Hauser, S, et al. DNA promoter methylation and ERG regulate the expression of CD24 in prostate cancer. Am J Pathol (2021) 191:618–30. doi:10.1016/j.ajpath.2020.12.014
105. Toghill, BJ, Saratzis, A, Harrison, SC, Verissimo, AR, Mallon, EB, and Bown, MJ. The potential role of DNA methylation in the pathogenesis of abdominal aortic aneurysm. Atherosclerosis (2015) 241:121–9. doi:10.1016/j.atherosclerosis.2015.05.001
106. Li, P, Newhardt, MF, Matsuzaki, S, Eyster, C, Pranay, A, Peelor, FF, et al. The loss of cardiac SIRT3 decreases metabolic flexibility and proteostasis in an age-dependent manner. Geroscience (2023) 45:983–99. doi:10.1007/s11357-022-00695-0
107. Jang, YC, Rodriguez, K, Lustgarten, MS, Muller, FL, Bhattacharya, A, Pierce, A, et al. Superoxide-mediated oxidative stress accelerates skeletal muscle atrophy by synchronous activation of proteolytic systems. Geroscience (2020) 42:1579–91. doi:10.1007/s11357-020-00200-5
108. Tomaru, U, Ito, T, Ohmura, Y, Higashikawa, K, Miyajima, S, Tomatsu, R, et al. Decreased proteasomal function induces neuronal loss and memory impairment. Am J Pathol (2021) 191:144–56. doi:10.1016/j.ajpath.2020.10.004
109. Gal, J, Katsumata, Y, Zhu, H, Srinivasan, S, Chen, J, Johnson, LA, et al. Apolipoprotein E proteinopathy is a major dementia-associated pathologic biomarker in individuals with or without the APOE epsilon 4 allele. Am J Pathol (2022) 192:564–78. doi:10.1016/j.ajpath.2021.11.013
110. Sabatini, DM. Twenty-five years of mTOR: uncovering the link from nutrients to growth. Proc Natl Acad Sci U S A (2017) 114:11818–25. doi:10.1073/pnas.1716173114
111. Teuliere, J, Bernard, C, Corel, E, Lapointe, FJ, Martens, J, Lopez, P, et al. Network analyses unveil ageing-associated pathways evolutionarily conserved from fungi to animals. Geroscience (2023) 45:1059–80. doi:10.1007/s11357-022-00704-2
112. Mota-Martorell, N, Jove, M, Pradas, I, Berdun, R, Sanchez, I, Naudi, A, et al. Gene expression and regulatory factors of the mechanistic target of rapamycin (mTOR) complex 1 predict mammalian longevity. Geroscience (2020) 42:1157–73. doi:10.1007/s11357-020-00210-3
113. Selvarani, R, Mohammed, S, and Richardson, A. Effect of rapamycin on aging and age-related diseases-past and future. Geroscience (2021) 43:1135–58. doi:10.1007/s11357-020-00274-1
114. Castro-Martinez, F, Candelario-Martinez, A, Encarnacion-Garcia, MR, Piedra-Quintero, Z, Bonilla-Moreno, R, Betanzos, A, et al. Rictor/Mammalian target of rapamycin complex 2 signaling protects colonocytes from apoptosis and prevents epithelial barrier breakdown. Am J Pathol (2021) 191:1537–49. doi:10.1016/j.ajpath.2021.06.004
115. Lee, HJ, Shin, DH, Song, JS, Park, JY, Kim, SY, Hwang, CS, et al. mTOR inhibition increases transcription factor E3 (TFE3) activity and modulates programmed death-ligand 1 (PD-L1) expression in translocation renal cell carcinoma. Am J Pathol (2021) 191:1999–2008. doi:10.1016/j.ajpath.2021.07.007
116. Lu, X, Peng, B, Chen, G, Pes, MG, Ribback, S, Ament, C, et al. YAP accelerates notch-driven cholangiocarcinogenesis via mTORC1 in mice. Am J Pathol (2021) 191:1651–67. doi:10.1016/j.ajpath.2021.05.017
117. Sahu, D, Huan, J, Wang, H, Sahoo, D, Casteel, DE, Klemke, RL, et al. Bladder cancer invasion is mediated by mammalian target of rapamycin complex 2-driven regulation of nitric oxide and invadopodia formation. Am J Pathol (2021) 191:2203–18. doi:10.1016/j.ajpath.2021.08.002
118. Wang, M, and Miller, RA. Fibroblasts from long-lived mutant mice exhibit increased autophagy and lower TOR activity after nutrient deprivation or oxidative stress. Aging Cell (2012) 11:668–74. doi:10.1111/j.1474-9726.2012.00833.x
119. LaRocca, TJ, Gioscia-Ryan, RA, Hearon, CM, and Seals, DR. The autophagy enhancer spermidine reverses arterial aging. Mech Ageing Dev (2013) 134:314–20. doi:10.1016/j.mad.2013.04.004
120. Kang, C, Xu, Q, Martin, TD, Li, MZ, Demaria, M, Aron, L, et al. The DNA damage response induces inflammation and senescence by inhibiting autophagy of GATA4. Science (2015) 349:aaa5612. doi:10.1126/science.aaa5612
121. Cuervo, AM, and Dice, JF. Age-related decline in chaperone-mediated autophagy. J Biol Chem (2000) 275:31505–13. doi:10.1074/jbc.M002102200
122. Tabibzadeh, S. Role of autophagy in aging: the good, the bad, and the ugly. Aging Cell (2023) 22:e13753. doi:10.1111/acel.13753
123. Martinez, RAS, Pinky, PD, Harlan, BA, and Brewer, GJ. GTP energy dependence of endocytosis and autophagy in the aging brain and Alzheimer's disease. Geroscience (2023) 45:757–80. doi:10.1007/s11357-022-00717-x
124. Kaushik, S, Tasset, I, Arias, E, Pampliega, O, Wong, E, Martinez-Vicente, M, et al. Autophagy and the hallmarks of aging. Ageing Res Rev (2021) 72:101468. doi:10.1016/j.arr.2021.101468
125. Aman, Y, Schmauck-Medina, T, Hansen, M, Morimoto, RI, Simon, AK, Bjedov, I, et al. Autophagy in healthy aging and disease. Nat Aging (2021) 1:634–50. doi:10.1038/s43587-021-00098-4
126. Zhang, K, Huang, Q, Peng, L, Lin, S, Liu, J, Zhang, J, et al. The multifunctional roles of autophagy in the innate immune response: implications for regulation of transplantation rejection. Front Cel Dev Biol (2022) 10:1007559. doi:10.3389/fcell.2022.1007559
127. Wu, YX, Jin, SH, and Cui, J. Autophagy and immune tolerance. Adv Exp Med Biol (2019) 1206:635–65. doi:10.1007/978-981-15-0602-4_28
128. Hashemi, M, Paskeh, MDA, Orouei, S, Abbasi, P, Khorrami, R, Dehghanpour, A, et al. Towards dual function of autophagy in breast cancer: a potent regulator of tumor progression and therapy response. Biomed Pharmacother (2023) 161:114546. doi:10.1016/j.biopha.2023.114546
129. Ballesteros-Alvarez, J, and Andersen, JKmTORC2. mTORC2: the other mTOR in autophagy regulation. Aging Cell (2021) 20:e13431. doi:10.1111/acel.13431
130. Al-Bari, MAA, and Xu, P. Molecular regulation of autophagy machinery by mTOR-dependent and -independent pathways. Ann N Y Acad Sci (2020) 1467:3–20. doi:10.1111/nyas.14305
131. Keller, CW, Adamopoulos, IE, and Lunemann, JD. Autophagy pathways in autoimmune diseases. J Autoimmun (2023) 136:103030. doi:10.1016/j.jaut.2023.103030
132. Zhou, L, Pinho, R, Gu, Y, and Radak, Z. The role of SIRT3 in exercise and aging. Cells (2022) 11:2596. doi:10.3390/cells11162596
133. Lautrup, S, Sinclair, DA, Mattson, MP, and Fang, EF. NAD(+) in brain aging and neurodegenerative disorders. Cell Metab (2019) 30:630–55. doi:10.1016/j.cmet.2019.09.001
134. Yoshino, J, Baur, JA, and Imai, SI. NAD(+) intermediates: the biology and therapeutic potential of NMN and NR. Cel Metab (2018) 27:513–28. doi:10.1016/j.cmet.2017.11.002
135. Rajman, L, Chwalek, K, and Sinclair, DA. Therapeutic potential of NAD-boosting molecules: the in vivo evidence. Cel Metab (2018) 27:529–47. doi:10.1016/j.cmet.2018.02.011
136. Ansari, A, Rahman, MS, Saha, SK, Saikot, FK, Deep, A, and Kim, KH. Function of the SIRT3 mitochondrial deacetylase in cellular physiology, cancer, and neurodegenerative disease. Aging Cell (2017) 16:4–16. doi:10.1111/acel.12538
137. Kitada, M, Ogura, Y, and Koya, D. The protective role of Sirt1 in vascular tissue: its relationship to vascular aging and atherosclerosis. Aging (Albany NY) (2016) 8:2290–307. doi:10.18632/aging.101068
138. Imai, SI, and Guarente, L. It takes two to tango: NAD(+) and sirtuins in aging/longevity control. NPJ Aging Mech Dis (2016) 2:16017. doi:10.1038/npjamd.2016.17
139. Houtkooper, RH, Pirinen, E, and Auwerx, J. Sirtuins as regulators of metabolism and healthspan. Nat Rev Mol Cel Biol (2012) 13:225–38. doi:10.1038/nrm3293
140. Chalkiadaki, A, and Guarente, L. Sirtuins mediate mammalian metabolic responses to nutrient availability. Nat Rev Endocrinol (2012) 8:287–96. doi:10.1038/nrendo.2011.225
141. Minor, RK, Baur, JA, Gomes, AP, Ward, TM, Csiszar, A, Mercken, EM, et al. SRT1720 improves survival and healthspan of obese mice. Sci Rep (2011) 1:70. doi:10.1038/srep00070
142. Csiszar, A, Labinskyy, N, Jimenez, R, Pinto, JT, Ballabh, P, Losonczy, G, et al. Anti-oxidative and anti-inflammatory vasoprotective effects of caloric restriction in aging: role of circulating factors and SIRT1. Mech Ageing Dev (2009) 130:518–27. doi:10.1016/j.mad.2009.06.004
143. Ferrara, N, Rinaldi, B, Corbi, G, Conti, V, Stiuso, P, Boccuti, S, et al. Exercise training promotes SIRT1 activity in aged rats. Rejuvenation Res (2008) 11:139–50. doi:10.1089/rej.2007.0576
144. Csiszar, A, Labinskyy, N, Podlutsky, A, Kaminski, PM, Wolin, MS, Zhang, C, et al. Vasoprotective effects of resveratrol and SIRT1: attenuation of cigarette smoke-induced oxidative stress and proinflammatory phenotypic alterations. Am J Physiol Heart Circ Physiol (2008) 294:H2721–35. doi:10.1152/ajpheart.00235.2008
145. Dasgupta, B, and Milbrandt, J. Resveratrol stimulates AMP kinase activity in neurons. Proc Natl Acad Sci USA (2007) 104:7217–22. doi:10.1073/pnas.0610068104
146. Alcendor, RR, Gao, S, Zhai, P, Zablocki, D, Holle, E, Yu, X, et al. Sirt1 regulates aging and resistance to oxidative stress in the heart. Circ Res (2007) 100:1512–21. doi:10.1161/01.RES.0000267723.65696.4a
147. Guarente, L. Calorie restriction and SIR2 genes--towards a mechanism. Mech Ageing Dev (2005) 126:923–8. doi:10.1016/j.mad.2005.03.013
148. Nemoto, S, Fergusson, MM, and Finkel, T. Nutrient availability regulates SIRT1 through a forkhead-dependent pathway. Science (2004) 306:2105–8. doi:10.1126/science.1101731
149. Cohen, HY, Miller, C, Bitterman, KJ, Wall, NR, Hekking, B, Kessler, B, et al. Calorie restriction promotes mammalian cell survival by inducing the SIRT1 deacetylase. Science (2004) 305:390–2. doi:10.1126/science.1099196
150. Howitz, KT, Bitterman, KJ, Cohen, HY, Lamming, DW, Lavu, S, Wood, JG, et al. Small molecule activators of sirtuins extend Saccharomyces cerevisiae lifespan. Nature (2003) 425:191–6. doi:10.1038/nature01960
151. You, Y, and Liang, W. SIRT1 and SIRT6: the role in aging-related diseases. Biochim Biophys Acta Mol Basis Dis (2023) 1869:166815. doi:10.1016/j.bbadis.2023.166815
152. Chen, C, Zhou, M, Ge, Y, and Wang, X. SIRT1 and aging related signaling pathways. Mech Ageing Dev (2020) 187:111215. doi:10.1016/j.mad.2020.111215
153. Raza, U, Tang, X, Liu, Z, and Liu, B. SIRT7: the seventh key to unlocking the mystery of aging. Physiol Rev (2024) 104:253–80. doi:10.1152/physrev.00044.2022
154. Li, H, and Cai, Z. SIRT3 regulates mitochondrial biogenesis in aging-related diseases. J Biomed Res (2022) 37:77–88. doi:10.7555/JBR.36.20220078
155. McDonnell, E, Peterson, BS, Bomze, HM, and Hirschey, MD. SIRT3 regulates progression and development of diseases of aging. Trends Endocrinol Metab (2015) 26:486–92. doi:10.1016/j.tem.2015.06.001
156. He, L, Liu, Q, Cheng, J, Cao, M, Zhang, S, Wan, X, et al. SIRT4 in ageing. Biogerontology (2023) 24:347–62. doi:10.1007/s10522-023-10022-5
157. Fabbrizi, E, Fiorentino, F, Carafa, V, Altucci, L, Mai, A, and Rotili, D. Emerging roles of SIRT5 in metabolism, cancer, and SARS-CoV-2 infection. Cells (2023) 12:852. doi:10.3390/cells12060852
158. Bringman-Rodenbarger, LR, Guo, AH, Lyssiotis, CA, and Lombard, DB. Emerging roles for SIRT5 in metabolism and cancer. Antioxid Redox Signal (2018) 28:677–90. doi:10.1089/ars.2017.7264
159. Wallace, DC. A mitochondrial paradigm of metabolic and degenerative diseases, aging, and cancer: a dawn for evolutionary medicine. Annu Rev Genet (2005) 39:359–407. doi:10.1146/annurev.genet.39.110304.095751
160. Schriner, SE, Linford, NJ, Martin, GM, Treuting, P, Ogburn, CE, Emond, M, et al. Extension of murine life span by overexpression of catalase targeted to mitochondria. Science (2005) 308:1909–11. doi:10.1126/science.1106653
161. Herbst, A, Prior, SJ, Lee, CC, Aiken, JM, McKenzie, D, Hoang, A, et al. Skeletal muscle mitochondrial DNA copy number and mitochondrial DNA deletion mutation frequency as predictors of physical performance in older men and women. Geroscience (2021) 43:1253–64. doi:10.1007/s11357-021-00351-z
162. Ahmed, NS, Foote, JB, and Singh, KK. Impaired mitochondria promote aging-associated sebaceous gland dysfunction and pathology. Am J Pathol (2022) 192:1546–58. doi:10.1016/j.ajpath.2022.07.006
163. Reiss, AB, Ahmed, S, Dayaramani, C, Glass, AD, Gomolin, IH, Pinkhasov, A, et al. The role of mitochondrial dysfunction in Alzheimer's disease: a potential pathway to treatment. Exp Gerontol (2022) 164:111828. doi:10.1016/j.exger.2022.111828
164. Springo, Z, Tarantini, S, Toth, P, Tucsek, Z, Koller, A, Sonntag, WE, et al. Aging exacerbates pressure-induced mitochondrial oxidative stress in mouse cerebral arteries. J Gerontol A Biol Sci Med Sci (2015) 70:1355–9. doi:10.1093/gerona/glu244
165. Tarantini, S, Valcarcel-Ares, NM, Yabluchanskiy, A, Fulop, GA, Hertelendy, P, Gautam, T, et al. Treatment with the mitochondrial-targeted antioxidant peptide SS-31 rescues neurovascular coupling responses and cerebrovascular endothelial function and improves cognition in aged mice. Aging Cell (2018) 17:e12731. doi:10.1111/acel.12731
166. Zampino, M, Spencer, RG, Fishbein, KW, Simonsick, EM, and Ferrucci, L. Cardiovascular health and mitochondrial function: testing an association. J Gerontol A Biol Sci Med Sci (2021) 76:361–7. doi:10.1093/gerona/glaa297
167. Saberi, M, Zhang, X, and Mobasheri, A. Targeting mitochondrial dysfunction with small molecules in intervertebral disc aging and degeneration. Geroscience (2021) 43:517–37. doi:10.1007/s11357-021-00341-1
168. Bua, E, Johnson, J, Herbst, A, Delong, B, McKenzie, D, Salamat, S, et al. Mitochondrial DNA-deletion mutations accumulate intracellularly to detrimental levels in aged human skeletal muscle fibers. Am J Hum Genet (2006) 79:469–80. doi:10.1086/507132
169. Swift, ML, Sell, C, and Azizkhan-Clifford, J. DNA damage-induced degradation of Sp1 promotes cellular senescence. Geroscience (2022) 44:683–98. doi:10.1007/s11357-021-00456-5
170. Han, X, Lei, Q, Xie, J, Liu, H, Li, J, Zhang, X, et al. Potential regulators of the senescence-associated secretory phenotype during senescence and aging. J Gerontol A Biol Sci Med Sci (2022) 77:2207–18. doi:10.1093/gerona/glac097
171. Matacchione, G, Perugini, J, Di Mercurio, E, Sabbatinelli, J, Prattichizzo, F, Senzacqua, M, et al. Senescent macrophages in the human adipose tissue as a source of inflammaging. Geroscience (2022) 44:1941–60. doi:10.1007/s11357-022-00536-0
172. Fielding, RA, Atkinson, EJ, Aversa, Z, White, TA, Heeren, AA, Achenbach, SJ, et al. Associations between biomarkers of cellular senescence and physical function in humans: observations from the lifestyle interventions for elders (LIFE) study. Geroscience (2022) 44:2757–70. doi:10.1007/s11357-022-00685-2
173. Kavanagh, K, Sherrill, C, Ruggiero, A, Block, M, Vemuri, R, Davis, M, et al. Biomarkers of senescence in non-human primate adipose depots relate to aging. Geroscience (2021) 43:343–52. doi:10.1007/s11357-020-00230-z
174. Karin, O, and Alon, U. Senescent cell accumulation mechanisms inferred from parabiosis. Geroscience (2021) 43:329–41. doi:10.1007/s11357-020-00286-x
175. Hense, JD, Garcia, DN, Isola, JV, Alvarado-Rincon, JA, Zanini, BM, Prosczek, JB, et al. Senolytic treatment reverses obesity-mediated senescent cell accumulation in the ovary. Geroscience (2022) 44:1747–59. doi:10.1007/s11357-022-00573-9
176. Dungan, CM, Figueiredo, VC, Wen, Y, VonLehmden, GL, Zdunek, CJ, Thomas, NT, et al. Senolytic treatment rescues blunted muscle hypertrophy in old mice. Geroscience (2022) 44:1925–40. doi:10.1007/s11357-022-00542-2
177. Iijima, H, Gilmer, G, Wang, K, Sivakumar, S, Evans, C, Matsui, Y, et al. Meta-analysis integrated with multi-omics data analysis to elucidate pathogenic mechanisms of age-related knee osteoarthritis in mice. J Gerontol A Biol Sci Med Sci (2022) 77:1321–34. doi:10.1093/gerona/glab386
178. Csipo, T, Lipecz, A, Ashpole, NM, Balasubramanian, P, and Tarantini, S. Astrocyte senescence contributes to cognitive decline. Geroscience (2020) 42:51–5. doi:10.1007/s11357-019-00140-9
179. Kiss, T, Nyul-Toth, A, Balasubramanian, P, Tarantini, S, Ahire, C, DelFavero, J, et al. Single-cell RNA sequencing identifies senescent cerebromicrovascular endothelial cells in the aged mouse brain. Geroscience (2020) 42:429–44. doi:10.1007/s11357-020-00177-1
180. Tarantini, S, Balasubramanian, P, Delfavero, J, Csipo, T, Yabluchanskiy, A, Kiss, T, et al. Treatment with the BCL-2/BCL-xL inhibitor senolytic drug ABT263/Navitoclax improves functional hyperemia in aged mice. Geroscience (2021) 43:2427–40. doi:10.1007/s11357-021-00440-z
181. Chae, JB, Jang, H, Son, C, Park, CW, Choi, H, Jin, S, et al. Targeting senescent retinal pigment epithelial cells facilitates retinal regeneration in mouse models of age-related macular degeneration. Geroscience (2021) 43:2809–33. doi:10.1007/s11357-021-00457-4
182. Park, JW, Park, JE, Kim, SR, Sim, MK, Kang, CM, and Kim, KS. Metformin alleviates ionizing radiation-induced senescence by restoring BARD1-mediated DNA repair in human aortic endothelial cells. Exp Gerontol (2022) 160:111706. doi:10.1016/j.exger.2022.111706
183. Yabluchanskiy, A, Tarantini, S, Balasubramanian, P, Kiss, T, Csipo, T, Fulop, GA, et al. Pharmacological or genetic depletion of senescent astrocytes prevents whole brain irradiation-induced impairment of neurovascular coupling responses protecting cognitive function in mice. Geroscience (2020) 42:409–28. doi:10.1007/s11357-020-00154-8
184. Sun, T, Zhang, L, Feng, J, Bao, L, Wang, J, Song, Z, et al. Characterization of cellular senescence in doxorubicin-induced aging mice. Exp Gerontol (2022) 163:111800. doi:10.1016/j.exger.2022.111800
185. Khadirnaikar, S, Chatterjee, A, and Shukla, S. Identification and characterization of senescence phenotype in lung adenocarcinoma with high drug sensitivity. Am J Pathol (2021) 191:1966–73. doi:10.1016/j.ajpath.2021.07.005
186. Aires, R, Porto, ML, de Assis, LM, Pereira, PAN, Carvalho, GR, Coco, LZ, et al. DNA damage and aging on hematopoietic stem cells: impact of oxidative stress in ApoE(-)/(-) mice. Exp Gerontol (2021) 156:111607. doi:10.1016/j.exger.2021.111607
187. Bencivenga, L, Strumia, M, Rolland, Y, Martinez, L, Cestac, P, Guyonnet, S, et al. Biomarkers of mitochondrial dysfunction and inflammaging in older adults and blood pressure variability. Geroscience (2023) 45:797–809. doi:10.1007/s11357-022-00697-y
188. Dugue, PA, Hodge, AM, Ulvik, A, Ueland, PM, Midttun, O, Rinaldi, S, et al. Association of markers of inflammation, the kynurenine pathway and B vitamins with age and mortality, and a signature of inflammaging. J Gerontol A Biol Sci Med Sci (2022) 77:826–36. doi:10.1093/gerona/glab163
189. Cribb, L, Hodge, AM, Yu, C, Li, SX, English, DR, Makalic, E, et al. Inflammation and epigenetic aging are largely independent markers of biological aging and mortality. J Gerontol A Biol Sci Med Sci (2022) 77:2378–86. doi:10.1093/gerona/glac147
190. Zamboni, M, Nori, N, Brunelli, A, and Zoico, E. How does adipose tissue contribute to inflammageing? Exp Gerontol (2021) 143:111162. doi:10.1016/j.exger.2020.111162
191. Sanfilippo, C, Castrogiovanni, P, Vinciguerra, M, Imbesi, R, Ulivieri, M, Fazio, F, et al. A sex-stratified analysis of neuroimmune gene expression signatures in Alzheimer's disease brains. Geroscience (2023) 45:523–41. doi:10.1007/s11357-022-00664-7
192. Kiss, T, Nyul-Toth, A, DelFavero, J, Balasubramanian, P, Tarantini, S, Faakye, J, et al. Spatial transcriptomic analysis reveals inflammatory foci defined by senescent cells in the white matter, hippocampi and cortical grey matter in the aged mouse brain. Geroscience (2022) 44:661–81. doi:10.1007/s11357-022-00521-7
193. Thadathil, N, Nicklas, EH, Mohammed, S, Lewis, TL, Richardson, A, and Deepa, SS. Necroptosis increases with age in the brain and contributes to age-related neuroinflammation. Geroscience (2021) 43:2345–61. doi:10.1007/s11357-021-00448-5
194. Mehdipour, M, Mehdipour, T, Skinner, CM, Wong, N, Liu, C, Chen, CC, et al. Plasma dilution improves cognition and attenuates neuroinflammation in old mice. Geroscience (2021) 43:1–18. doi:10.1007/s11357-020-00297-8
195. Liang, Z, Zhang, T, Liu, H, Li, Z, Peng, L, Wang, C, et al. Inflammaging: the ground for sarcopenia? Exp Gerontol (2022) 168:111931. doi:10.1016/j.exger.2022.111931
196. Luo, J, le Cessie, S, Blauw, GJ, Franceschi, C, Noordam, R, and van Heemst, D. Systemic inflammatory markers in relation to cognitive function and measures of brain atrophy: a Mendelian randomization study. Geroscience (2022) 44:2259–70. doi:10.1007/s11357-022-00602-7
197. Lu, WH, Giudici, KV, Morley, JE, Guyonnet, S, Parini, A, Aggarwal, G, et al. Investigating the combination of plasma amyloid-beta and geroscience biomarkers on the incidence of clinically meaningful cognitive decline in older adults. Geroscience (2022) 44:1489–503. doi:10.1007/s11357-022-00554-y
198. Jimenez, L, Silva, A, Calissi, G, Grenho, I, Monteiro, R, Mayoral-Varo, V, et al. Screening health-promoting compounds for their capacity to induce the activity of FOXO3. J Gerontol A Biol Sci Med Sci (2022) 77:1485–93. doi:10.1093/gerona/glab265
199. Li, S, Hou, Y, Liu, K, Zhu, H, Qiao, M, Sun, X, et al. Metformin protects against inflammation, oxidative stress to delay poly I:C-induced aging-like phenomena in the gut of an annual fish. J Gerontol A Biol Sci Med Sci (2022) 77:276–82. doi:10.1093/gerona/glab298
200. Brazao, V, Colato, RP, Santello, FH, Duarte, A, Goulart, A, Sampaio, PA, et al. Melatonin regulates antioxidant defense and inflammatory response by activating Nrf2-dependent mechanisms and inhibiting NFkappaB expression in middle-aged T. cruzi infected rats. Exp Gerontol (2022) 167:111895. doi:10.1016/j.exger.2022.111895
201. Caldeira, CA, Santos, MA, Araujo, GR, Lara, RC, Franco, FN, and Chaves, MM. Resveratrol: change of SIRT 1 and AMPK signaling pattern during the aging process. Exp Gerontol (2021) 146:111226. doi:10.1016/j.exger.2021.111226
202. Gregory, S, Pullen, H, Ritchie, CW, Shannon, OM, Stevenson, EJ, and Muniz-Terrera, G. Mediterranean diet and structural neuroimaging biomarkers of Alzheimer's and cerebrovascular disease: a systematic review. Exp Gerontol (2023) 172:112065. doi:10.1016/j.exger.2022.112065
203. Chenhuichen, C, Cabello-Olmo, M, Barajas, M, Izquierdo, M, Ramirez-Velez, R, Zambom-Ferraresi, F, et al. Impact of probiotics and prebiotics in the modulation of the major events of the aging process: a systematic review of randomized controlled trials. Exp Gerontol (2022) 164:111809. doi:10.1016/j.exger.2022.111809
204. Ezzati, A, and Pak, VM. The effects of time-restricted eating on sleep, cognitive decline, and Alzheimer's disease. Exp Gerontol (2023) 171:112033. doi:10.1016/j.exger.2022.112033
205. Ramaker, ME, Corcoran, DL, Apsley, AT, Kobor, MS, Kraus, VB, Kraus, WE, et al. Epigenome-wide association study analysis of calorie restriction in humans, CALERIETM trial analysis. J Gerontol A Biol Sci Med Sci (2022) 77:2395–401. doi:10.1093/gerona/glac168
206. Fassier, P, Kang, JH, Lee, IM, Grodstein, F, and Vercambre, MN. Vigorous Physical Activity and Cognitive Trajectory Later in Life: prospective Association and Interaction by Apolipoprotein E e4 in the Nurses' Health Study. J Gerontol A Biol Sci Med Sci (2022) 77:817–25. doi:10.1093/gerona/glab169
207. Gudiksen, A, Qoqaj, A, Ringholm, S, Wojtaszewski, J, Plomgaard, P, and Pilegaard, H. Ameliorating effects of lifelong physical activity on healthy aging and mitochondrial function in human white adipose tissue. J Gerontol A Biol Sci Med Sci (2022) 77:1101–11. doi:10.1093/gerona/glab356
208. Romashkan, S, Chang, H, and Hadley, EC. National institute on aging workshop: repurposing drugs or dietary supplements for their senolytic or senomorphic effects: considerations for clinical trials. J Gerontol A Biol Sci Med Sci (2021) 76:1144–52. doi:10.1093/gerona/glab028
209. Saccon, TD, Nagpal, R, Yadav, H, Cavalcante, MB, Nunes, ADC, Schneider, A, et al. Senolytic combination of dasatinib and quercetin alleviates intestinal senescence and inflammation and modulates the gut microbiome in aged mice. J Gerontol A Biol Sci Med Sci (2021) 76:1895–905. doi:10.1093/gerona/glab002
210. Kowald, A, and Kirkwood, TBL. Senolytics and the compression of late-life mortality. Exp Gerontol (2021) 155:111588. doi:10.1016/j.exger.2021.111588
211. Whitson, JA, Johnson, R, Wang, L, Bammler, TK, Imai, SI, Zhang, H, et al. Age-related disruption of the proteome and acetylome in mouse hearts is associated with loss of function and attenuated by elamipretide (SS-31) and nicotinamide mononucleotide (NMN) treatment. Geroscience (2022) 44:1621–39. doi:10.1007/s11357-022-00564-w
212. Whitson, JA, Martin-Perez, M, Zhang, T, Gaffrey, MJ, Merrihew, GE, Huang, E, et al. Elamipretide (SS-31) treatment attenuates age-associated post-translational modifications of heart proteins. Geroscience (2021) 43:2395–412. doi:10.1007/s11357-021-00447-6
213. Alfaras, I, Di Germanio, C, Bernier, M, Csiszar, A, Ungvari, Z, Lakatta, EG, et al. Pharmacological strategies to retard cardiovascular aging. Circ Res (2016) 118:1626–42. doi:10.1161/CIRCRESAHA.116.307475
214. Mitchell, SJ, Martin-Montalvo, A, Mercken, EM, Palacios, HH, Ward, TM, Abulwerdi, G, et al. The SIRT1 activator SRT1720 extends lifespan and improves health of mice fed a standard diet. Cell Rep (2014) 6:836–43. doi:10.1016/j.celrep.2014.01.031
215. Hubbard, BP, and Sinclair, DA. Small molecule SIRT1 activators for the treatment of aging and age-related diseases. Trends Pharmacol Sci (2014) 35:146–54. doi:10.1016/j.tips.2013.12.004
216. Kiss, T, Balasubramanian, P, Valcarcel-Ares, MN, Tarantini, S, Yabluchanskiy, A, Csipo, T, et al. Nicotinamide mononucleotide (NMN) treatment attenuates oxidative stress and rescues angiogenic capacity in aged cerebromicrovascular endothelial cells: a potential mechanism for the prevention of vascular cognitive impairment. Gero Sci (2019) 41:619–30. doi:10.1007/s11357-019-00074-2
217. Kiss, T, Giles, CB, Tarantini, S, Yabluchanskiy, A, Balasubramanian, P, Gautam, T, et al. Nicotinamide mononucleotide (NMN) supplementation promotes anti-aging miRNA expression profile in the aorta of aged mice, predicting epigenetic rejuvenation and anti-atherogenic effects. Geroscience (2019) 41:419–39. doi:10.1007/s11357-019-00095-x
218. Tarantini, S, Valcarcel-Ares, MN, Toth, P, Yabluchanskiy, A, Tucsek, Z, Kiss, T, et al. Nicotinamide mononucleotide (NMN) supplementation rescues cerebromicrovascular endothelial function and neurovascular coupling responses and improves cognitive function in aged mice. Redox Biol (2019) 24:101192. doi:10.1016/j.redox.2019.101192
219. Csiszar, A, Tarantini, S, Yabluchanskiy, A, Balasubramanian, P, Kiss, T, Farkas, E, et al. Role of endothelial NAD+ deficiency in age-related vascular dysfunction. Am J Physiol Heart Circ Physiol (2019) 316:H1253–H1266. doi:10.1152/ajpheart.00039.2019
220. Kiss, T, Nyul-Toth, A, Balasubramanian, P, Tarantini, S, Ahire, C, Yabluchanskiy, A, et al. Nicotinamide mononucleotide (NMN) supplementation promotes neurovascular rejuvenation in aged mice: transcriptional footprint of SIRT1 activation, mitochondrial protection, anti-inflammatory, and anti-apoptotic effects. Geroscience (2020) 42:527–46. doi:10.1007/s11357-020-00165-5
221. Tarantini, S, Yabluchanskiy, A, Csipo, T, Fulop, G, Kiss, T, Balasubramanian, P, et al. Treatment with the poly(ADP-ribose) polymerase inhibitor PJ-34 improves cerebromicrovascular endothelial function, neurovascular coupling responses and cognitive performance in aged mice, supporting the NAD+ depletion hypothesis of neurovascular aging. Geroscience (2019) 41:533–42. doi:10.1007/s11357-019-00101-2
222. Das, A, Huang, GX, Bonkowski, MS, Longchamp, A, Li, C, Schultz, MB, et al. Impairment of an endothelial NAD(+)-H2S signaling network is a reversible cause of vascular aging. Cell (2018) 173:74–89. doi:10.1016/j.cell.2018.02.008
223. Clement, J, Wong, M, Poljak, A, Sachdev, P, and Braidy, N. The plasma NAD(+) metabolome is dysregulated in "normal" aging. Rejuvenation Res (2018) 22:121–30. doi:10.1089/rej.2018.2077
224. Mills, KF, Yoshida, S, Stein, LR, Grozio, A, Kubota, S, Sasaki, Y, et al. Long-Term administration of nicotinamide mononucleotide mitigates age-associated physiological decline in mice. Cel Metab (2016) 24:795–806. doi:10.1016/j.cmet.2016.09.013
225. de Picciotto, NE, Gano, LB, Johnson, LC, Martens, CR, Sindler, AL, Mills, KF, et al. Nicotinamide mononucleotide supplementation reverses vascular dysfunction and oxidative stress with aging in mice. Aging Cell (2016) 15:522–30. doi:10.1111/acel.12461
226. Araki, T, Sasaki, Y, and Milbrandt, J. Increased nuclear NAD biosynthesis and SIRT1 activation prevent axonal degeneration. Science (2004) 305:1010–3. doi:10.1126/science.1098014
227. Blair, RV, Vaccari, M, Doyle-Meyers, LA, Roy, CJ, Russell-Lodrigue, K, Fahlberg, M, et al. Acute respiratory distress in aged, SARS-CoV-2-infected african green monkeys but not rhesus macaques. Am J Pathol (2021) 191:274–82. doi:10.1016/j.ajpath.2020.10.016
228. Mulka, KR, Beck, SE, Solis, CV, Johanson, AL, Queen, SE, McCarron, ME, et al. Progression and resolution of severe acute respiratory syndrome coronavirus 2 (SARS-CoV-2) infection in golden Syrian hamsters. Am J Pathol (2022) 192:195–207. doi:10.1016/j.ajpath.2021.10.009
229. Gustine, JN, and Jones, D. Immunopathology of hyperinflammation in COVID-19. Am J Pathol (2021) 191:4–17. doi:10.1016/j.ajpath.2020.08.009
230. Johnson, JE, McGuone, D, Xu, ML, Jane-Wit, D, Mitchell, RN, Libby, P, et al. Coronavirus disease 2019 (COVID-19) coronary vascular thrombosis: correlation with neutrophil but not endothelial activation. Am J Pathol (2022) 192:112–20. doi:10.1016/j.ajpath.2021.09.004
231. Martin, TR. Lung injury and repair in coronavirus disease 2019-related acute lung injury. Am J Pathol (2022) 192:406–9. doi:10.1016/j.ajpath.2022.01.001
232. Nicosia, RF, Ligresti, G, Caporarello, N, Akilesh, S, and Ribatti, D. COVID-19 vasculopathy: mounting evidence for an indirect mechanism of endothelial injury. Am J Pathol (2021) 191:1374–84. doi:10.1016/j.ajpath.2021.05.007
233. Pujadas, E, Beaumont, M, Shah, H, Schrode, N, Francoeur, N, Shroff, S, et al. Molecular profiling of coronavirus disease 2019 (COVID-19) autopsies uncovers novel disease mechanisms. Am J Pathol (2021) 191:2064–71. doi:10.1016/j.ajpath.2021.08.009
234. Salazar, E, Christensen, PA, Graviss, EA, Nguyen, DT, Castillo, B, Chen, J, et al. Significantly decreased mortality in a large cohort of coronavirus disease 2019 (COVID-19) patients transfused early with convalescent plasma containing high-titer anti-severe acute respiratory syndrome coronavirus 2 (SARS-CoV-2) spike protein IgG. Am J Pathol (2021) 191:90–107. doi:10.1016/j.ajpath.2020.10.008
235. Ting, C, Aspal, M, Vaishampayan, N, Huang, SK, Riemondy, KA, Wang, F, et al. Fatal COVID-19 and non-COVID-19 acute respiratory distress syndrome is associated with incomplete alveolar type 1 epithelial cell differentiation from the transitional state without fibrosis. Am J Pathol (2022) 192:454–67. doi:10.1016/j.ajpath.2021.11.014
236. Whitmore, HAB, and Kim, LA. Understanding the role of blood vessels in the neurologic manifestations of coronavirus disease 2019 (COVID-19). Am J Pathol (2021) 191:1946–54. doi:10.1016/j.ajpath.2021.04.017
237. Fagyas, M, Nagy, B, Raduly, AP, Manyine, IS, Martha, L, Erdosi, G, et al. The majority of severe COVID-19 patients develop anti-cardiac autoantibodies. Geroscience (2022) 44:2347–60. doi:10.1007/s11357-022-00649-6
238. Moccia, F, Gerbino, A, Lionetti, V, Miragoli, M, Munaron, LM, Pagliaro, P, et al. COVID-19-associated cardiovascular morbidity in older adults: a position paper from the Italian Society of Cardiovascular Researches. Geroscience (2020) 42:1021–49. doi:10.1007/s11357-020-00198-w
239. Danics, K, Pesti, A, Toro, K, Kiss-Dala, N, Szlavik, J, Lakatos, B, et al. A COVID-19-association-dependent categorization of death causes in 100 autopsy cases. Geroscience (2021) 43:2265–87. doi:10.1007/s11357-021-00451-w
240. Peterfi, A, Meszaros, A, Szarvas, Z, Penzes, M, Fekete, M, Feher, A, et al. Comorbidities and increased mortality of COVID-19 among the elderly: a systematic review. Physiol Int (2022) 109:163–76. doi:10.1556/2060.2022.00206
241. Gado, K, Kovacs, AK, Domjan, G, Nagy, ZZ, and Bednarik, GD. COVID-19 and the elderly. Physiol Int (2022) 109:177–85. doi:10.1556/2060.2022.00203
242. Bello-Chavolla, OY, Gonzalez-Diaz, A, Antonio-Villa, NE, Fermin-Martinez, CA, Marquez-Salinas, A, Vargas-Vazquez, A, et al. Unequal impact of structural health determinants and comorbidity on COVID-19 severity and lethality in older Mexican adults: considerations beyond chronological aging. J Gerontol A Biol Sci Med Sci (2021) 76:e52–e59. doi:10.1093/gerona/glaa163
243. Esme, M, Koca, M, Dikmeer, A, Balci, C, Ata, N, Dogu, BB, et al. Older adults with coronavirus disease 2019: a nationwide study in Turkey. J Gerontol A Biol Sci Med Sci (2021) 76:e68–e75. doi:10.1093/gerona/glaa219
244. Farrelly, C. The COVID-19 pandemic, biogerontology, and the ageing of humanity. J Gerontol A Biol Sci Med Sci (2021) 76:e92–e96. doi:10.1093/gerona/glab027
245. Kuo, CL, Pilling, LC, Atkins, JL, Masoli, JAH, Delgado, J, Tignanelli, C, et al. Biological aging predicts vulnerability to COVID-19 severity in UK biobank participants. J Gerontol A Biol Sci Med Sci (2021) 76:e133–e141. doi:10.1093/gerona/glab060
246. Marengoni, A, Zucchelli, A, Vetrano, DL, Armellini, A, Botteri, E, Nicosia, F, et al. Beyond chronological age: frailty and multimorbidity predict in-hospital mortality in patients with coronavirus disease 2019. J Gerontol A Biol Sci Med Sci (2021) 76:e38–e45. doi:10.1093/gerona/glaa291
247. Marquez-Salinas, A, Fermin-Martinez, CA, Antonio-Villa, NE, Vargas-Vazquez, A, Guerra, EC, Campos-Munoz, A, et al. Adaptive metabolic and inflammatory responses identified using accelerated aging metrics are linked to adverse outcomes in severe SARS-CoV-2 infection. J Gerontol A Biol Sci Med Sci (2021) 76:e117–e126. doi:10.1093/gerona/glab078
248. Mendes, A, Herrmann, FR, Perivier, S, Gold, G, Graf, CE, and Zekry, D. Delirium in older patients with COVID-19: prevalence, risk factors, and clinical relevance. J Gerontol A Biol Sci Med Sci (2021) 76:e142–e146. doi:10.1093/gerona/glab039
249. Poco, PCE, Aliberti, MJR, Dias, MB, Takahashi, SF, Leonel, FC, Altona, M, et al. Divergent: age, frailty, and atypical presentations of COVID-19 in hospitalized patients. J Gerontol A Biol Sci Med Sci (2021) 76:e46–e51. doi:10.1093/gerona/glaa280
250. Zerah, L, Baudouin, E, Pepin, M, Mary, M, Krypciak, S, Bianco, C, et al. Clinical characteristics and outcomes of 821 older patients with SARS-cov-2 infection admitted to acute care geriatric wards. J Gerontol A Biol Sci Med Sci (2021) 76:e4–e12. doi:10.1093/gerona/glaa210
251. Penfold, RS, Zazzara, MB, Osterdahl, MF, Welch, C, Ni Lochlainn, M, Freidin, MB, et al. Individual factors including age, BMI, and heritable factors underlie temperature variation in sickness and in health: an observational, multi-cohort study. J Gerontol A Biol Sci Med Sci (2022) 77:1890–7. doi:10.1093/gerona/glab295
252. Tisminetzky, M, Delude, C, Hebert, T, Carr, C, Goldberg, RJ, and Gurwitz, JH. Age, multiple chronic conditions, and COVID-19: a literature review. J Gerontol A Biol Sci Med Sci (2022) 77:872–8. doi:10.1093/gerona/glaa320
253. VoPham, T, Harris, HR, Tinker, LF, Manson, JE, Meliker, JR, Wassertheil-Smoller, S, et al. The impact of the COVID-19 pandemic on older women in the women's health initiative. J Gerontol A Biol Sci Med Sci (2022) 77:S3–S12. doi:10.1093/gerona/glac056
254. Farshbafnadi, M, Kamali Zonouzi, S, Sabahi, M, Dolatshahi, M, and Aarabi, MH. Aging and COVID-19 susceptibility, disease severity, and clinical outcomes: the role of entangled risk factors. Exp Gerontol (2021) 154:111507. doi:10.1016/j.exger.2021.111507
255. Nidadavolu, LS, and Walston, JD. Underlying vulnerabilities to the cytokine storm and adverse COVID-19 outcomes in the aging immune system. J Gerontol A Biol Sci Med Sci (2021) 76:e13–e18. doi:10.1093/gerona/glaa209
256. Zhou, J, Sun, Y, Huang, W, and Ye, K. Altered blood cell traits underlie a major genetic locus of severe COVID-19. J Gerontol A Biol Sci Med Sci (2021) 76:e147–e154. doi:10.1093/gerona/glab035
257. Garcia-Torre, A, Bueno-Garcia, E, Lopez-Martinez, R, Rioseras, B, Moro-Garcia, MA, Alonso-Alvarez, S, et al. Surviving older patients show preserved cellular and humoral immunological memory several months after SARS-CoV-2 infection. J Gerontol A Biol Sci Med Sci (2022) 77:33–40. doi:10.1093/gerona/glab206
258. Justice, JN, Gubbi, S, Kulkarni, AS, Bartley, JM, Kuchel, GA, and Barzilai, N. A geroscience perspective on immune resilience and infectious diseases: a potential case for metformin. Geroscience (2021) 43:1093–112. doi:10.1007/s11357-020-00261-6
259. Pence, BD. Severe COVID-19 and aging: are monocytes the key? Geroscience (2020) 42:1051–61. doi:10.1007/s11357-020-00213-0
260. Xu, K, Wei, Y, Giunta, S, Zhou, M, and Xia, S. Do inflammaging and coagul-aging play a role as conditions contributing to the co-occurrence of the severe hyper-inflammatory state and deadly coagulopathy during COVID-19 in older people? Exp Gerontol (2021) 151:111423. doi:10.1016/j.exger.2021.111423
261. Fekete, M, Szarvas, Z, Fazekas-Pongor, V, Feher, A, Dosa, N, Lehoczki, A, et al. COVID-19 infection in patients with chronic obstructive pulmonary disease: from pathophysiology to therapy. Mini-review. Physiol Int (2022) 109:9–19. doi:10.1556/2060.2022.00172
262. Feher, A, Szarvas, Z, Lehoczki, A, Fekete, M, and Fazekas-Pongor, V. Co-infections in COVID-19 patients and correlation with mortality rate. Minireview. Physiol Int (2022) 109:1–8. doi:10.1556/2060.2022.00015
263. Kohli, J, Campisi, J, and Demaria, M. A novel suicide gene therapy for the treatment of p16(Ink4a)-overexpressing tumors. Oncotarget (2018) 9:7274–81. doi:10.18632/oncotarget.23752
264. Abdul-Aziz, AM, Sun, Y, Hellmich, C, Marlein, CR, Mistry, J, Forde, E, et al. Acute myeloid leukemia induces protumoral p16INK4a-driven senescence in the bone marrow microenvironment. Blood (2019) 133:446–56. doi:10.1182/blood-2018-04-845420
265. Patil, P, Dong, Q, Wang, D, Chang, J, Wiley, C, Demaria, M, et al. Systemic clearance of p16(INK4a) -positive senescent cells mitigates age-associated intervertebral disc degeneration. Aging Cell (2019) 18:e12927. doi:10.1111/acel.12927
266. Jeon, OH, Kim, C, Laberge, RM, Demaria, M, Rathod, S, Vasserot, AP, et al. Local clearance of senescent cells attenuates the development of post-traumatic osteoarthritis and creates a pro-regenerative environment. Nat Med (2017) 23:775–81. doi:10.1038/nm.4324
267. Kim, HN, Chang, J, Iyer, S, Han, L, Campisi, J, Manolagas, SC, et al. Elimination of senescent osteoclast progenitors has no effect on the age-associated loss of bone mass in mice. Aging Cell (2019) 18:e12923. doi:10.1111/acel.12923
268. Istvan, L, Czako, C, Elo, A, Mihaly, Z, Sotonyi, P, Varga, A, et al. Imaging retinal microvascular manifestations of carotid artery disease in older adults: from diagnosis of ocular complications to understanding microvascular contributions to cognitive impairment. Geroscience (2021) 43:1703–23. doi:10.1007/s11357-021-00392-4
269. Czako, C, Kovacs, T, Ungvari, Z, Csiszar, A, Yabluchanskiy, A, Conley, S, et al. Retinal biomarkers for Alzheimer's disease and vascular cognitive impairment and dementia (VCID): implication for early diagnosis and prognosis. Geroscience (2020) 42:1499–525. doi:10.1007/s11357-020-00252-7
270. Drewelies, J, Hueluer, G, Duezel, S, Vetter, VM, Pawelec, G, Steinhagen-Thiessen, E, et al. Using blood test parameters to define biological age among older adults: association with morbidity and mortality independent of chronological age validated in two separate birth cohorts. Geroscience (2022) 44:2685–99. doi:10.1007/s11357-022-00662-9
271. Cummings, SR, and Kritchevsky, SB. Endpoints for geroscience clinical trials: health outcomes, biomarkers, and biologic age. Geroscience (2022) 44:2925–31. doi:10.1007/s11357-022-00671-8
272. Amgalan, A, Maher, AS, Ghosh, S, Chui, HC, Bogdan, P, and Irimia, A. Brain age estimation reveals older adults' accelerated senescence after traumatic brain injury. Geroscience (2022) 44:2509–25. doi:10.1007/s11357-022-00597-1
273. Kwon, D, and Belsky, DW. A toolkit for quantification of biological age from blood chemistry and organ function test data: BioAge. Geroscience (2021) 43:2795–808. doi:10.1007/s11357-021-00480-5
274. Crimmins, EM, Thyagarajan, B, Kim, JK, Weir, D, and Faul, J. Quest for a summary measure of biological age: the health and retirement study. Geroscience (2021) 43:395–408. doi:10.1007/s11357-021-00325-1
275. Chan, MS, Arnold, M, Offer, A, Hammami, I, Mafham, M, Armitage, J, et al. A biomarker-based biological age in UK biobank: composition and prediction of mortality and hospital admissions. J Gerontol A Biol Sci Med Sci (2021) 76:1295–302. doi:10.1093/gerona/glab069
276. Horvath, S, Lin, DTS, Kobor, MS, Zoller, JA, Said, JW, Morgello, S, et al. HIV, pathology and epigenetic age acceleration in different human tissues. Geroscience (2022) 44:1609–20. doi:10.1007/s11357-022-00560-0
277. Nwanaji-Enwerem, JC, Colicino, E, Gao, X, Wang, C, Vokonas, P, Boyer, EW, et al. Associations of plasma folate and vitamin B6 with blood DNA methylation age: an analysis of one-carbon metabolites in the VA normative aging study. J Gerontol A Biol Sci Med Sci (2021) 76:760–9. doi:10.1093/gerona/glaa257
278. Kresovich, JK, Martinez Lopez, AM, Garval, EL, Xu, Z, White, AJ, Sandler, DP, et al. Alcohol consumption and methylation-based measures of biological age. J Gerontol A Biol Sci Med Sci (2021) 76:2107–11. doi:10.1093/gerona/glab149
279. Crimmins, EM, Thyagarajan, B, Levine, ME, Weir, DR, and Faul, J. Associations of age, sex, race/ethnicity, and education with 13 epigenetic clocks in a nationally representative U.S. Sample: the health and retirement study. J Gerontol A Biol Sci Med Sci (2021) 76:1117–23. doi:10.1093/gerona/glab016
280. Vetter, VM, Kalies, CH, Sommerer, Y, Spira, D, Drewelies, J, Regitz-Zagrosek, V, et al. Relationship between 5 epigenetic clocks, telomere length, and functional capacity assessed in older adults: cross-sectional and longitudinal analyses. J Gerontol A Biol Sci Med Sci (2022) 77:1724–33. doi:10.1093/gerona/glab381
281. McCrory, C, Fiorito, G, Hernandez, B, Polidoro, S, O'Halloran, AM, Hever, A, et al. GrimAge outperforms other epigenetic clocks in the prediction of age-related clinical phenotypes and all-cause mortality. J Gerontol A Biol Sci Med Sci (2021) 76:741–9. doi:10.1093/gerona/glaa286
282. Van Remmen, H, Freeman, WM, Miller, BF, Kinter, M, Wren, JD, Chiao, A, et al. Oklahoma Nathan Shock Aging Center - assessing the basic biology of aging from genetics to protein and function. Geroscience (2021) 43:2183–203. doi:10.1007/s11357-021-00454-7
283. Shadel, GS, Adams, PD, Berggren, WT, Diedrich, JK, Diffenderfer, KE, Gage, FH, et al. The San Diego Nathan Shock Center: tackling the heterogeneity of aging. Geroscience (2021) 43:2139–48. doi:10.1007/s11357-021-00426-x
284. Salmon, AB, Nelson, JF, Gelfond, JAL, Javors, M, Ginsburg, B, Lopez-Cruzan, M, et al. San Antonio Nathan Shock Center: your one-stop shop for aging research. Geroscience (2021) 43:2105–18. doi:10.1007/s11357-021-00417-y
285. Korstanje, R, Peters, LL, Robinson, LL, Krasinski, SD, and Churchill, GA. The jackson laboratory nathan shock center: impact of genetic diversity on aging. Geroscience (2021) 43:2129–37. doi:10.1007/s11357-021-00421-2
286. Kaeberlein, M, Bitto, A, Dunham, MJ, Ladiges, W, Lee, SI, MacCoss, MJ, et al. University of Washington Nathan Shock Center: innovation to advance aging research. Geroscience (2021) 43:2161–5. doi:10.1007/s11357-021-00413-2
287. Curran, SP, Lithgow, GJ, Verdin, E, and Cohen, P. University of Southern California and buck institute nathan shock center: multidimensional models of aging. Geroscience (2021) 43:2119–27. doi:10.1007/s11357-021-00416-z
288. Cuervo, AM, Huffman, DM, Vijg, J, Milman, S, Singh, R, and Barzilai, N. Einstein-Nathan shock center: translating the hallmarks of aging to extend human health span. Geroscience (2021) 43:2167–82. doi:10.1007/s11357-021-00428-9
289. Austad, SN, van der Willik, O, Lederman, S, and Kerr, C. The nathan shock centers. Geroscience (2021) 43:2103–4. doi:10.1007/s11357-021-00425-y
Keywords: geroscience, senescence, cancer, cardiovascular disease, ageing
Citation: Fekete M, Major D, Feher A, Fazekas-Pongor V and Lehoczki A (2024) Geroscience and pathology: a new frontier in understanding age-related diseases. Pathol. Oncol. Res. 30:1611623. doi: 10.3389/pore.2024.1611623
Received: 04 December 2023; Accepted: 07 February 2024;
Published: 23 February 2024.
Edited by:
Andrea Ladányi, National Institute of Oncology (NIO), HungaryCopyright © 2024 Fekete, Major, Feher, Fazekas-Pongor and Lehoczki. This is an open-access article distributed under the terms of the Creative Commons Attribution License (CC BY). The use, distribution or reproduction in other forums is permitted, provided the original author(s) and the copyright owner(s) are credited and that the original publication in this journal is cited, in accordance with accepted academic practice. No use, distribution or reproduction is permitted which does not comply with these terms.
*Correspondence: Vince Fazekas-Pongor, pongor.vince@semmelweis.hu